Fig. 1.
Attenuation correction in PET/MRI. The attenuation map is generated using fat and water images from a DIXON magnetic resonance sequence. The -map only differentiates between air, lung, fat, and soft tissue. Using this -map NAC (non-attenuation-corrected) PET images are then corrected according to the respective tissue-specific attenuation coefficients
Another source of photon scatter and attenuation in PET/MRI are artificial components outside the body but within the FOV, such as coils, patient monitoring devices, positioning aids, earphones, cables, and the patient bed itself. For the MRI system these objects are “invisible” and thus do not contribute to the -map. Only the patient bed and the fixed coils are implanted in the precalculated attenuation models [25]. Consequently, vendors have redesigned their hardware, although the effects are still detectable. Whether there is a relevant impact on diagnostic scans is not yet known [26].
Cardiac Devices
Cardiac devices, such as pacemakers, implantable cardioverter defibrillators (ICDs), or cardiac resynchronization therapy (CRT), cause artifacts on CT that result in an overestimation of the attenuation. The effect on the quantification of tracer uptake seems to be negligible in PET/CT [27]. Analogously, non-magnetic metals cause a signal void in MRI that exceeds the actual size of the object, possibly resulting in an underestimation of the attenuation. More importantly, these devices may interact with the radiofrequency, which may result in their malfunction or heating. Therefore, many subjects cannot be examined using MRI (and thus by PET/MRI); this is a growing problem because the number of patients with diseases qualifying for the implantation of such cardiac devices is increasing [28, 29]. Phase analysis using nuclear medicine studies (such as PET or SPECT) has been proposed to better identify patients who might respond to CRT implantation [30, 31]; however, larger clinical trials are still warranted.
Workflows for Cardiac PET/MRI
As mentioned above, attenuation correction is a crucial aspect of imaging and so is the exact alignment of the – map and the acquired PET data. One major advantage of MRI over CT is that the attenuation correction scan may be repeated as often as necessary without any radiation. Unfortunately, the subsequent necessary choice of the ideal µ-map is a time-consuming, inconvenient matter. According to our experience, however, in the vast majority of cases there is satisfactory alignment between PET data and the µ-map.
Another important consideration regarding PET/MRI is that most data are acquired in “parallel” and not truly “simultaneously”. PET acquires a volume with frame lengths ranging from a few seconds up to 20–30 min (or more) depending on the chosen protocol (tracer, half-life, injected activity, endurance of the patient, etc.). Consequently, the acquired PET data comprise motion that took place during the scan (e.g., heart beat, ventilation and “true” patient motion) whereas in MR data are usually acquired sequentially (even in 3D acquisitions), with acquisition times ranging from about 50 ms for dynamic scans to several breath-holds for high-resolution images. A major problem with sequential imaging is that the data are acquired over several breath-holds; since the reproducibility of breath-holding is limited, this results in partially overlapping (or partially lacking) data. However, the imaging of moving objects with MRI would result in enormous, unacceptable artifacts. With cardiac PET/MRI, this raises the issue, that ungated (cardiac cycle and ventilation) PET images are compared to or fused with, for example, enddiastolic magnetic resonance images that are acquired during breath-hold. Another inconvenient and time-consuming matter is that the PET volume data have to be coregistered with the MRI data in various (partially overlapping) slices of various positions (e.g., short axes; two-, three-, and four-chamber view). However, researchers are aware of this problem and techniques such as motion-triggered acquisition [32] and software corrections [33] are being developed that allow free-breathing during imaging.
Still, parallel PET/MRI is much more favorable than sequential PET/MRI. The sequential approach (either on separate scanners or on scanners connected via a common rail system) is not only inconvenient for the patient and personnel; it is also logistically demanding. Parallel PET/MRI, on the other hand, can not only improve patient compliance and comfort, it can also increase patient throughput and thus costefficiency. Two potential work flows are depicted in Fig. 2.
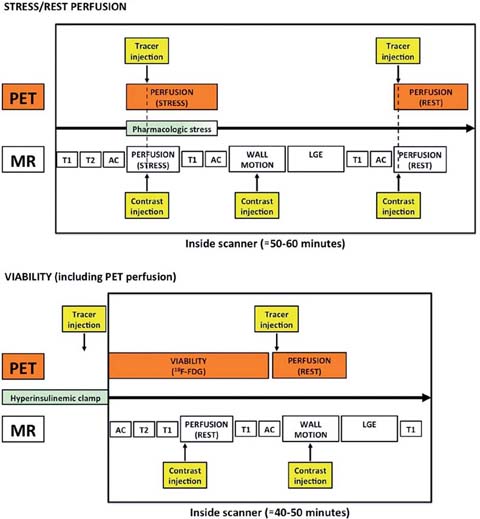
Fig. 2.
Two potential workflows (stress/rest perfusion, viability including perfusion) for simultaneous PET/MRI
An application that might benefit from the truly simultaneous acquisition of PET and MRI data is myocardial perfusion imaging (MPI). The initial benefit would be the capability to cross-validate one modality with the other (which is of research interest). Taking into consideration PET, with its vast variety of specific tracers and its good volume-coverage, and MRI, with its high inplane resolution, a synergistic effect would, for example, be the separate investigation of epicardial and endocardial perfusion. However, some hurdles still need to be overcome: (1) MRI usually only acquires a few slices of the heart, (2) Gdchelate based contrast medium, used in most applications, has properties that are unfavorable for truly quantitative perfusion assessment, such as a low extraction fraction and a non-specific (only partially perfusion-dependent) uptake by the myocardium. The major advantage is, however, that PET and MRI data can be acquired under truly identical conditions, even though the injection speed of the imaging agent (PET: ≈30 s, MRI: ≈5 s) as well as the acquisition time (MRI: ≈1 min, PET: ≈10 min) differ significantly.
Cardiovascular Applications Using Hybrid PET/MRI
Myocardial Perfusion Imaging
The diagnosis of flow-limiting coronary artery disease (CAD) and the investigation of the hemodynamic significance of known CAD are the most regularly conducted studies in nuclear cardiology, due to its high sensitivity and specificity [34] and its high value in patient management [35–37]. PET MPI helps in clinical decision-making as the extent of ischemic tissue determined by this imaging modality allows patients to be assigned to optimal therapy, either revascularization or medical therapy [38]. Furthermore, there is increasing evidence that PET MPI offers advantages over SPECT MPI due to the absolute quantification of myocardial blood flow (MBF), attenuation correction, and superior image quality, especially in obese patients [39]. So far, several PET perfusion tracers have been established: N-13 ammonia, O-15 water, rubidium-82 (Rb-82), and F-18 flurpiridaz. The relatively short half-lives of N-13 ammonia (≈10 min), O-15 water (122 s) and Rb-82 (76 s) allow rapid PET MPI with only short lag times between the stress and the at-rest studies. The disadvantages of these tracers are, however, the high positron energies, resulting in decreased image quality (Rb-82), the need of an on-site cyclotron (N-13 ammonia, O-15 water) or of a generator, which is only cost-effective when a certain patient-throughput can be guaranteed (Rb-82), or the fact that stress imaging is only feasible when the patient is administered the pharmacological agent and the tracer while inside the scanner (N-13 ammonia, O-15 water, Rb-82). F-18 flurpiridaz offers some advantages, e.g. a low positron energy, a half-life that is long enough to enable tracer distribution even to hospitals and clinics in rural areas, the option of ergometer exercise with tracer injection outside the scanner, and possibly a simplified MBF estimation approach [40, 41]. Despite the above-mentioned advantages of PET MPI and its use in many centers, MRI has also been evaluated recently for the purpose of MPI. The underlying principle that first-pass MRI with gadolinium-based contrast agents (e.g., Gd-DTPA) can be used to detect CAD was shown about 20 years ago [42]; since then, a multitude of studies have investigated its value in flow-limiting CAD [43, 44]. While these studies consisted almost exclusively of visual analysis, the absolute quantification of MBF is known to be of great value in patients suffering from extensive CAD and balanced ischemia [45, 46]. The feasibility of quantifying MBF using MRI has been investigated. One study evaluated a rather simple approach, namely, the up-slope ratio as an index of coronary flow reserve [47]. However, in a subsequent study, this approach was definitively shown to result in an underestimation of the flow reserve when compared to N-13 ammonia PET MPI [48]. Subsequently, a more complex model, using the central volume principle, was investigated for absolute MBF quantification [49]. This approach was recently studied in 41 patients who underwent PET and MRI MPI separately [50]. Good agreement for the coronary flow reserve between these two modalities was obtained; the correlation was weak for absolute MBF values, though. It should be noted, however, that a rare quantification method for N-13 ammonia was applied [51] and that differences in absolute MBF may also be due to the fact that Gd-chelates do not enter the cells and thus show a distribution only in the interstitial space (also in increased interstitial spaces such as scarred myocardium) [52].
There are patients in whom even extremely well-tolerated MRI contrast agents cannot be used and alternative approaches, without the use of any contrast medium, are being developed. For example, arterial spin labeling (ASL), which originates from techniques to determine cerebral blood flow, has been investigated in a preclinical study, where it demonstrated a good correlation with the results of O-15 water PET [53]. In patients, ASL was able to measure the increase in blood flow induced by adenosine [54]. The obvious advantages of ASL are the possibility of repetitive and continuous measurements of MBF without need for any contrast agents
In summary, cardiac MPI using simultaneous PET/MRI might be valuable to detect subendocardial ischemia by MRI and to quantify absolute MBF using PET.
Myocardial Viability Imaging
Another potential application of PET/MR in cardiology is myocardial viability imaging. Hypoperfused myocardium exhibits a shift of its metabolism from fatty acids towards glucose, a state called “hibernation” [55]. Several studies have shown that myocardium in this state is prone to recover after revascularization [56]. Usually, FDG PET is the method of choice for non-invasive viability imaging [55]. Recently, cardiac MRI using the late gadolinium enhancement (LGE) technique has emerged as a possible alternative. Notably, however, unlike FDG PET, this approach images non-viable, scarred myocardium, information used to draw conclusions regarding the potential of non-scarred myocardium to recover. The enrichment of Gd-chelates in increased extracellular space (such as scarred myocardium) results in a reduced wash-out of the contrast agent compared to remote myocardium which may be visualized in cardiac MRI using inversion recovery sequences, in which the signal of the remote myocardium is nulled [57]. Despite the fundamental difference in these approaches (imaging viable vs. non-viable tissue) there is good agreement between the two modalities [58]. One essential property of MRI is its high in-plane resolution (1–3 mm), which enables the differentiation between transmural (>50%) and non-transmural (<50%) scarred myocardium [59]. Another interesting finding is that in patients with suspected CAD but with-out known myocardial infarction, even small areas of scarred myocardium carry prognostic significance [60]. A similar observation was described in a study comparing areas of non-transmural LGE with SPECT MPI [61]. Since small areas of infarction may be overlooked by PET because of partial volume effects, this fact is relevant in hybrid PET/MRI, as an improved tissue classification can be expected. Our group made similar observations in patients shortly after myocardial infarction, in whom severely reduced FDG uptake was accompanied by non-transmural LGE signal only (Fig. 3). Accordingly, hybrid PET/MRI might ultimately result in an improved prediction of wall motion recovery after revascularization, when information such as wall motion and thickening, FDG uptake, perfusion, and LGE transmurality are integrated.
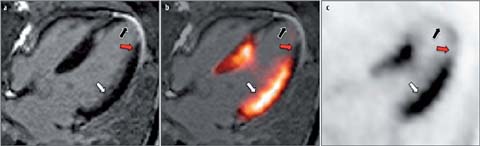
Fig. 3 a–c.
FDG PET/MRI “viability” imaging shortly after acute myocardial infarction (MI). Example from a patient who was imaged a few days after acute MI by FDG PET/MRI (hyperinsulinemic/euglycemic clamp). Four-chamber views of late gadolinium enhancement (LGE) MRI (a), FDG PET (c), and fusion of LGE MRI and FDG PET (b) are depicted. Early after acute MI, different patterns of FDG uptake and LGE transmurality can be observed: (1) normal FDG uptake and no LGE (white arrows), (2) reduced/absent FDG uptake and transmural LGE (black arrows), and (3) reduced FDG uptake and non-transmural LGE (red arrows)
As mentioned above, the assessment of global and regional wall motion is a relevant surrogate marker for myocardial vitality and therapy response after revascularization. Several studies have shown that left ventricular parameters, such as ejection fraction, end-systolic volume, and end-diastolic volume carry prognostic significance. MRI is the gold standard for the assessment of these parameters and it has been extensively validated [62]. The disadvantages of MRI regarding the assessment of left ventricular function are, however, that data are acquired in multiple slices in short- and long axis orientations (usually in 20–30 phases) and thus do not provide fully volumetric data. Gated PET, however, is fully volumetric and acquired at typically 8–12 phases; it has been validated in patients with and without cardiac diseases [63, 64]. Furthermore, gated PET can be performed in patients regardless of implanted cardiac devices. Another fact worth noting is that gated PET data are analyzed using highly automated software, which results in high intra- and interobserver reproducibility, whereas MRI usually requires at least some manual interaction for segmentation of the myocardium.
Atherosclerotic Plaque Imaging
While coronary artery and plaque imaging are still the domain of CT angiography, there is increasing interest in the use of MRI in this setting. Besides the fact that MR angiography can be performed without any radiation whereas the radiation dose of CT angiography, depending on the protocol and scanner, is ≈1–20 mSv [65, 66]. Moreover, MRI can provide additional valuable information, such as characterization of the vessel wall or the plaque itself [67, 68]. An interesting finding by our group was the observation of Gd-contrast enhancement in the vessel wall following myocardial infarction that correlated with the degree of stenosis; the enhancement resolved in a 3-month follow-up scan, possibly representing reduced inflammatory activity [69]. Also, several PET tracers have been used to image atherosclerotic plaques, especially FDG. It is assumed that an increased FDG up-take is related to an intense macrophage infiltration, representing a high vulnerability [70, 71]. Another tracer that has recently been investigated in 80 patients with myocardial infarction or stable angina is F-18 fluoride (F-I8 NaF) [72]. In 93% of the patients with myocardial infarction, F-18 NaF uptake was highest in the culprit lesion whereas FDG uptake was obscured by the myocardial uptake. Furthermore, 45% of the patients with stable angina had plaques with focal F-18 NaF uptake that were associated with high-risk features on intravascular ultrasound. However, whether this tracer can be useful to improve patient management remains to be determined in future studies. Potentially valuable tracers targeting integrins, such as V 3, have also been investigated in preclinical models and are currently being translated into clinical applications [73–75]. Because tracer uptake in atherosclerotic plaques and the vessel wall is usually rather low, cardiac and respiratory motion is an issue, which might ultimately be addressed using MRI. Hybrid PET/MRI might help to make molecular PET imaging of the coronary arteries and culprit lesions feasible, through the use of novel tracers.
Conclusion
For a long time, the integration of PET and MRI seemed practically impossible. Accordingly, following the recent introduction of hybrid PET/MRI systems, expectations were high. Yet, almost 4 years after the introduction of the first clinically available hybrid PET/MRI scanner, studies with a cardiovascular focus are scarce. The main advantages of this novel technique are the reduction of the radiation dose to the patient, increased patient comfort and throughput compared to sequential imaging, and the capability to simultaneously investigate biological processes under the same physiological conditions. The major downside is a complex workflow requiring additional personnel and training, which results in extra costs. In summary, PET/MRI systems represent a superb research tool, and first studies demonstrating their additional value in clinical routine are being conducted.
References
1.
Kajander S, Ukkonen H, Sipila H et al (2009) Low radiation dose imaging of myocardial perfusion and coronary angiography with a hybrid PET/CT scanner. Clinical physiology and functional imaging 29:81–88.CrossRefPubMedCentralPubMed
2.
Zaidi H, Ojha N, Morich M et al (2011) Design and performance evaluation of a whole-body Ingenuity TF PET-MRI sys-tem. Physics in medicine and biology 56:3091–3106.CrossRefPubMedCentralPubMed
3.
Delso G, Furst S, Jakoby B et al (2011) Performance measurements of the Siemens mMR integrated whole-body PET/MR scanner. J Nucl Med 52:1914–1922.CrossRefPubMed
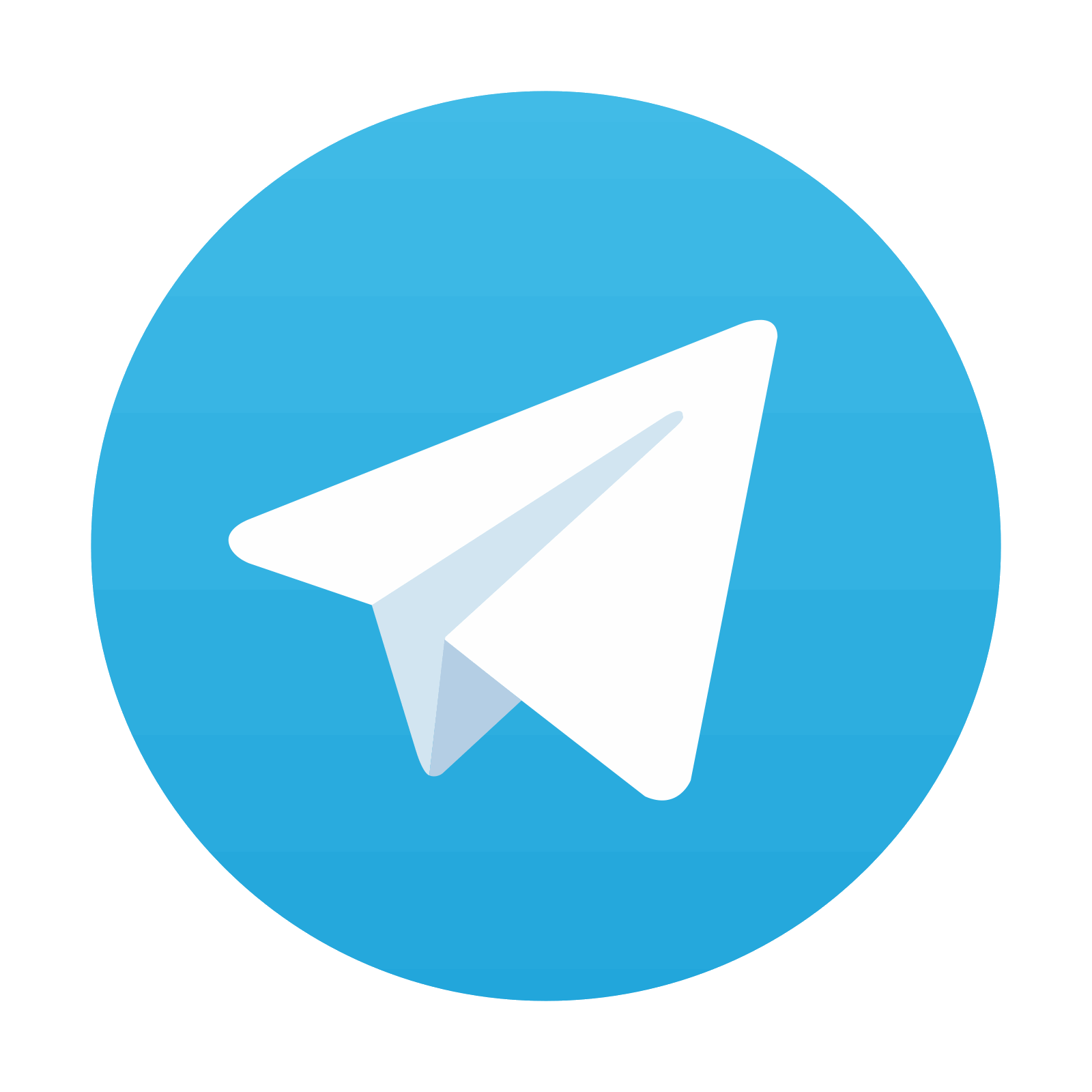
Stay updated, free articles. Join our Telegram channel
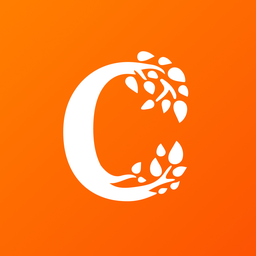
Full access? Get Clinical Tree
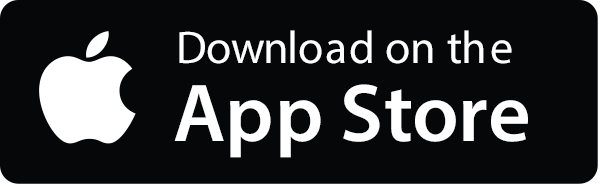
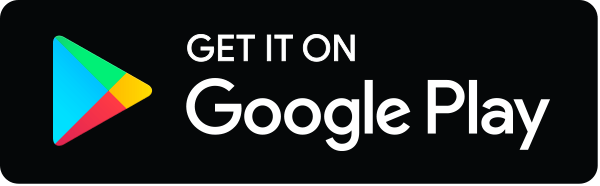