Virend K. Somers *
Cardiovascular Manifestations of Autonomic Disorders
Cardiovascular function is closely linked and responsive to numerous endogenous and exogenous factors. This interplay is mediated through rapid and often subtle neurohormonal changes. One of the most important mechanisms whereby rapid circulatory control is achieved is the autonomic nervous system, which modulates cardiac function through direct effects on the heart and vascular tone.
Overview of Neural Circulatory Control
The autonomic nervous system can be subdivided into sympathetic, parasympathetic, and enteric components. The principal cardiovascular influences are mediated through the sympathetic and parasympathetic systems. The interplay between these two systems and their relative balance help determine cardiovascular responses under a variety of conditions. These responses usually take the form of changes in blood pressure (BP) or heart rate. Knowledge of the physiology whereby BP and heart rate react to autonomic modulation is important for understanding how disorders of the autonomic nervous system can affect cardiovascular function.
Baroreceptors
Autonomic responses, capillary shift mechanisms, hormonal responses, and kidney and fluid balance mechanisms all interact to maintain control of BP. Of these, the autonomic nervous system offers the most rapid response system. Neural circulatory regulation occurs via increased contractility of the heart or vasoconstriction of the arterial or venous circulations in response to information received from baroreceptors. This afferent information is synthesized and integrated and appropriate responses generated in the vasomotor center of the brain.
Arterial Baroreceptors
Arterial baroreceptors are located in the carotid sinuses, in the aortic arch, and at the origin of the right subclavian artery. The carotid sinus baroreceptors are innervated by the glossopharyngeal nerve (cranial nerve IX), and the aortic arch baroreceptors are innervated by the vagus nerve (cranial nerve X). Baroreceptors are stretch-dependent mechanoreceptors that sense changes in pressure, which is transmitted via afferents to the nucleus tractus solitarius (NTS) in the brainstem. When distended, the baroreceptors are activated and generate action potentials that increase in frequency in correlation to the amount of stretch. Thus spike frequency is used as a surrogate measure of BP at the level of the NTS, with higher frequency correlating with higher BP.
Cardiopulmonary Baroreceptors
Although arterial baroreceptors are the most sensitive receptors, low-pressure receptors in the heart and venae cavae termed cardiopulmonary receptors also play a role in modulation of BP. They respond primarily to changes in volume but also to chemical stimuli. They project via vagal afferents to the NTS and via spinal sympathetic afferents to the spinal cord. Stimulation results in vasodilation and inhibition of vasopressin release. Furthermore, a stretch stimulus depresses renal sympathetic nerve activity and has been shown to play a role in modulating renin release, thereby resulting in diuresis and natriuresis and thus regulating whole-body fluid volume to maintain BP homeostasis.1 The cardiopulmonary receptors have only limited direct influence on control of the heart rate.
Breathing and Chemoreflexes
The chemoreflexes are modulators of sympathetic activation and play an important role in cardiovascular autonomic tone. The chemoreceptors are most simply divided into their central and peripheral components. Peripheral chemoreceptors are located in the carotid bodies and respond to hypoxemia, whereas central chemoreceptors are located in the brainstem and respond mostly to hypercapnia. Hypoxemia or hypercapnia results in hyperventilation and vascular sympathetic activation. Inhibitory influences on the chemoreflex drive are seen with stretch of the pulmonary afferents and with activation of the baroreflex, both of which have a greater influence on peripheral than on central chemoreflexes.
Diving Reflex
Under circumstances of prolonged apnea, a unique state of simultaneous increased parasympathetic drive to the heart and increased sympathetic drive to the vasculature occurs (Fig. 89-1). This state is seen in diving mammals and sometimes in humans during prolonged submersion in water. In response to hypoxia, the body usually seeks to increase ventilation and blood flow to end organs to maintain tissue oxygenation. However, in response to prolonged hypoxia in the absence of breathing, the body no longer experiences replenishment of oxygen stores and the normal homeostatic mechanisms alter to maintain oxygen delivery to organs vital to life—the brain and heart. This is achieved by decreasing oxygen delivery to much of the rest of the body via increased sympathetic vasoconstriction. This increased sympathetic outflow, however, does not constrict the cerebral vasculature because cerebral vascular tone is under autoregulatory control. Furthermore, myocardial oxygen demand is decreased because of bradycardia caused by an increase in parasympathetic tone. Thus, it is possible for individuals under exceptional circumstances to survive for prolonged periods of up to 5 minutes or longer under anoxic conditions.
Autonomic Testing
Experimental evidence of an association between lethal arrhythmias and increased sympathetic or reduced vagal activity has spurred the development of several quantitative markers of autonomic activity. Generally, the two easiest and most economic means of studying the interplay between autonomic and cardiac function are via orthostatics and the Valsalva maneuver, although both are nonspecific. When a patient is evaluated for syncope, a cost-effective means of exploring a neurocardiogenic cause is with a tilt-table test (see Chapters 34 and 40). Furthermore, studies of baroreflex sensitivity, heart rate variability, heart rate recovery, and chemoreflexes have been shown to be of assistance in direct assessment of autonomic dysfunction. Finally, blood levels of norepinephrine and its metabolites may assist in discriminating between different types of dysautonomia.
Orthostatics
Orthostatic hypotension (OH) is defined as a decrease of more than 20 mm Hg in systolic pressure or a decrease of more than 10 mm Hg in diastolic pressure after rising to a standing position from a supine position. BP and heart rate should be measured after symptoms develop or once 3 minutes has passed after rising to the standing position. If the patient is unable to stand, orthostatics may be done after the patient has risen to a sitting position with the feet dangling over the edge of the bed. OH is an inability to maintain sufficient BP and adequate cerebral perfusion against gravity. Generally, on arising from a supine position, an average person may lose about 700 mL of blood from the thorax. This results in decreased stroke volume, as well as decreased systolic pressure and increased diastolic pressure. Compensation occurs via an increase in heart rate and slight peripheral vasoconstriction. In individuals intolerant of orthostasis, venous pooling secondary to decreased muscle and vascular tone and decreased circulating blood volume may develop in response to standing. When testing orthostatics it is important to note that a significant decrease in BP without a corresponding rise in heart rate suggests abnormal autonomic innervation to the heart and may represent an underlying neuropathy, chronotropic incompetence, or drug therapy that blunts the heart rate response, such as beta blockade.
Valsalva Maneuver
The Valsalva maneuver becomes useful for testing patients at the bedside when done in conjunction with continuous electrocardiographic monitoring. During monitoring the patient blows continuously into a closed system for 12 seconds at 40 mm Hg, and the fastest heart rate during the maneuver is divided by the slowest heart rate immediately afterward. A quotient of less than 1.4 is suggestive of autonomic impairment. However, this is nonspecific. Recovery of BP after the Valsalva maneuver may provide a useful measure of adrenergic vasoconstrictor reserve (see later). The systolic BP (SBP) response to the Valsalva maneuver has four phases. Phase 1 apparently increases BP with onset of the Valsalva maneuver, and phase 2 represents normalization of BP during a sustained Valsalva maneuver. Phase 3 represents a dip after Valsalva release, and Phase 4 involves an “overshoot” several seconds later. In patients with pulmonary hypertension, a prompt fall in SBP during phase 2 suggests normal pulmonary artery wedge pressure, whereas sustained elevation denotes left-sided congestion, thus providing noninvasive insight into the hemodynamic basis of pulmonary hypertension.2 Measurement of left ventricular outflow tract obstruction via the Valsalva maneuver has been proposed as a reliable means of evaluating the indication for percutaneous transluminal septal myocardial ablation, as well as for assessment of treatment effect.3 A “sympathetic index” derived from the change in BP at baseline and the level during phase 2 has been suggested as a useful sign of OH and sympathetic failure (see later).4
Other Tests of Autonomic Function
Baroreflex Sensitivity
Testing baroreflex control of the heart rate involves measuring the reflex increase in the R-R interval in response to an increase in BP. The increase in BP has historically been achieved by use of an alpha-adrenergic agonist, most often phenylephrine. Intravenous injection of a bolus of phenylephrine induces a 20– to 30–mm Hg increase in SBP. Generally, a linear relationship exists between the increase in the R-R interval and the increase in SBP. The slope is used to quantify the sensitivity of the arterial baroreflex; it is typically steep in healthy individuals but decreases with advancing age and flattens even more with severe cardiovascular disease, such as hypertension or heart failure.
As a measure of autonomic function, baroreflex sensitivity decreases (i.e., shows a flatter slope) with sympathetic dominance and increases (i.e., shows a steeper slope) with parasympathetic dominance. In the search for noninvasive ways to measure baroreflex sensitivity, various devices and maneuvers have been used. The FINAPRES (from finger arterial pressure) device is one such example and has been used in the ATRAMI (Autonomic Tone and Reflexes After Myocardial Infarction) study. Spontaneous increases and decreases in BP, as well as associated changes in the R-R interval, have been used to determine the spontaneous baroreflex. Furthermore, spectral techniques for analyzing the relationship between beat-to-beat oscillations in BP and R-R intervals are being studied as possible alternatives to the more invasive method of phenylephrine infusion.
Heart Rate Variability
Heart rate variability has become a commonly used but difficult-to-interpret means of studying the interplay between the autonomic nervous system and cardiovascular function. The phenomenon being measured in heart rate variability is oscillation in the interval between consecutive heartbeats, as well as the variance in heart rates.
Actual measurement of heart rate variability has been achieved via multiple different modalities, most notably by using time domain and frequency domain methods. It is usually calculated by analyzing the time series of beat-to-beat intervals from electrocardiographic or arterial pressure tracings. A simple example of time domain measurement of heart rate variability is calculation of the standard deviation of beat-to-beat intervals. The time domain graph of a value shows how the signal varies over time. The frequency domain graph, however, shows how much of a signal lies within given frequency bands over a range of frequencies. It involves the use of mathematical transforms, such as the Fourier transform, to decompose a function into an infinite or finite number of frequencies. Spectral density analysis is the most common frequency domain method used and involves measurement of how the power of a signal or time series is distributed at any particular frequency.
The usefulness of heart rate variability as a measure of autonomic function and as a predictor of mortality has been suggested by a number of studies. In the 1970s, Wolf and colleagues showed a higher risk for postinfarction mortality with reduced heart rate variability, and Ewing and associates developed simple bedside tests that use short-term differences in the R-R interval as a means of detecting autonomic neuropathy in diabetics. In the late 1980s, heart rate variability was shown to be an independent predictor of post–myocardial infarction mortality. Altered heart rate variability has been associated with other pathologic conditions such as hypertension, hemorrhagic shock, and septic shock and has been accepted as an independent predictor of mortality after myocardial infarction.6
Heart rate turbulence, thought to be a reflection of baroreflex sensitivity, may also provide prognostically useful information. Abnormal heart rate turbulence has been linked to total mortality and sudden death in patients after myocardial infarction and those with heart failure7 and may be useful in predicting arrhythmic risk in heart failure patients with a left ventricular ejection fraction higher than 30%.8 In diabetes, abnormal heart rate variability can be used to help identify cardiovascular autonomic neuropathy (see later), which is also accompanied by increased risk for mortality.9 Sudden death is the major cause of cardiac mortality in patients with end-stage renal disease (ESRD). Emerging evidence suggests that impairments in heart rate variability and baroreflex function may help identify patients with ESRD at greatest cardiac risk.10
Heart Rate Recovery
During exercise the heart rate rises, initially secondary to a reduction in vagal tone and then because of increased sympathetic activity. After exercise, parasympathetic reactivation and reduced sympathetic activity contribute to the recovery of resting heart rate. The rate at which the heart rate returns to baseline, measured over the first minute after exercise, is termed heart rate recovery. Delayed heart rate recovery is a marker of decreased vagal activity, which has been shown to be an independent risk factor for sudden cardiac death.11 Patients with heart failure and a preserved ejection fraction, in contrast to hypertensive and healthy controls, have impaired heart rate recovery, as well as chronotropic incompetence during maximal exercise.12 Postexercise heart rate recovery may also be a useful tool in screening for cardiac autonomic neuropathy in patients with type 2 diabetes (see later).13
Tilt-Table Testing
Tilt-table testing is often conducted in patients with a history of syncope to diagnose possible dysautonomic causes of syncope (see Chapter 40). The test is considered positive if the patient experiences symptoms associated with a drop in BP or an arrhythmia. These abnormalities are suggestive of dysfunction of the autonomic system. Normally, BP will compensate via an increase in heart rate and constriction of blood vessels in the legs. In some patients, fainting or syncope could be associated with a precipitous drop in BP (vasodepressor syncope) or pulse rate (cardioinhibitory syncope) or a mixed response, thus requiring continuous monitoring of both.
Orthostatic Hypotension
OH (not the same as orthostatic intolerance; see later) is secondary to neurogenic and non-neurogenic conditions.14 Neurogenic causes are addressed later (see Autonomic Dysregulation). Non-neurogenic causes include hypovolemia, cardiac dysfunction, and medications (including those used to treat hypertension, myocardial ischemia, depression, psychosis, and Alzheimer and Parkinson disease). OH and tachycardia may also occur after prolonged bed rest or following exposure to microgravity, such as in space flight. In some patients, OH may be accompanied by fatigue, cognitive dysfunction, and emotional difficulties, as well as by abnormalities in gait and falls.15,16 Symptoms can be debilitating and confine patients to bed; the consequent physical deconditioning may worsen the overall problem. Longitudinal studies have suggested that OH can increase the risk for stroke, myocardial ischemia, and mortality (see later). The therapeutic goal is to attenuate or eliminate symptoms rather than restore normotension. Pharmacologic therapy is often suboptimal and should be combined with interventions such as compression of venous capacitance beds, use of physical countermaneuvers, and intermittent water bolus treatment. Treatment can be difficult, and the development of supine hypertension should be minimized, especially in patients with diabetes, heart failure, or cardiac ischemia.
However, although symptoms of OH may include dizziness and syncope, asymptomatic OH is far more common and represents an independent risk factor for mortality and cardiovascular disease.15 In a prospective study of more than 33,000 individuals, OH was present in over 6% and was associated with age, female sex, hypertension, antihypertension treatment, increased heart rate, diabetes, low body mass index, and recurrent smoking.16 Those with OH had significantly greater risk for all-cause mortality, especially those younger than 42 years, and higher risk for coronary events.16
Autonomic Dysregulation
Dysautonomias refer to any dysfunction of the autonomic nervous system, whether central, peripheral, or secondary to other disease processes. In general, the most common dysautonomias are those affecting the sympathetic system. However, the parasympathetic system and conditions of increased parasympathetic tone, such as during sleep or with endurance training, are also important to understand because they can have significant implications for cardiovascular health.
The sympathetic dysautonomias are the most common and may be characterized by disorders of release, function, or reuptake of norepinephrine, the main sympathetic chemical messenger. Furthermore, altered local blood flow and clearance of norepinephrine from the circulation can be manifested as a dysautonomia. The sympathetic dysautonomias can generally be divided into two groups—those associated with decreased function, in which orthostasis often occurs, and those associated with increased outflow, in which hypertension and/or tachycardia may be present.
Primary Chronic Autonomic Failure
Orthostatic intolerance is a key manifestation of neurocirculatory failure. It often serves as the initial symptom. However, not all OH is symptomatic of neurocirculatory failure. Most cases of OH result from blood loss, volume depletion, or a prolonged bedridden state. Only rarely does it result from true autonomic failure.
Chronic autonomic failure is distinguishable from acute-onset autonomic dysfunction syndromes by its progressive nature and prognosis. Generally, chronic autonomic failure can be subdivided into secondary and primary failure, with secondary failure being far more common. In cases of secondary failure, the cause is usually clear and treatment involves therapy for the underlying disorder. However, when autonomic failure dominates the clinical findings and a clear cause is not apparent, it is termed primary chronic autonomic failure.1
Pure Autonomic Failure
Pure autonomic failure involves OH in the absence of symptoms or signs of central neurodegeneration. Thus the dysfunction occurs at the level of peripheral neurons and not in the central nervous system. The functional error lies in available levels of norepinephrine, which are low when supine and rise minimally with standing.1 OH and an inadequate chronotropic response to standing and to the Valsalva maneuver are therefore evident. No direct effect on longevity occurs in these patients.
Multisystem Atrophy
MSA includes autonomic failure with signs and symptoms of progressive central neurodegeneration. It is generally divided into parkinsonian, cerebellar, and mixed forms. Symptoms develop in the sixth or seventh decade of life, and patients exhibit sympathetic and parasympathetic dysfunction. In addition to OH, findings may include impotence, loss of sweating, abnormal pupillary responses, reduced intraocular pressure, and urinary incontinence. Alveolar hypoventilation, central sleep apnea, and other breathing abnormalities may be present. However, in a study of patients with MSA, ventilatory responses to hypoxia and hypercapnia during wakefulness were preserved despite the presence of autonomic failure and impaired cardiovascular responses to these stimuli.17 Patients with MSA are known to have neuropathologic evidence of neurodegeneration in putative chemosensitive areas,18
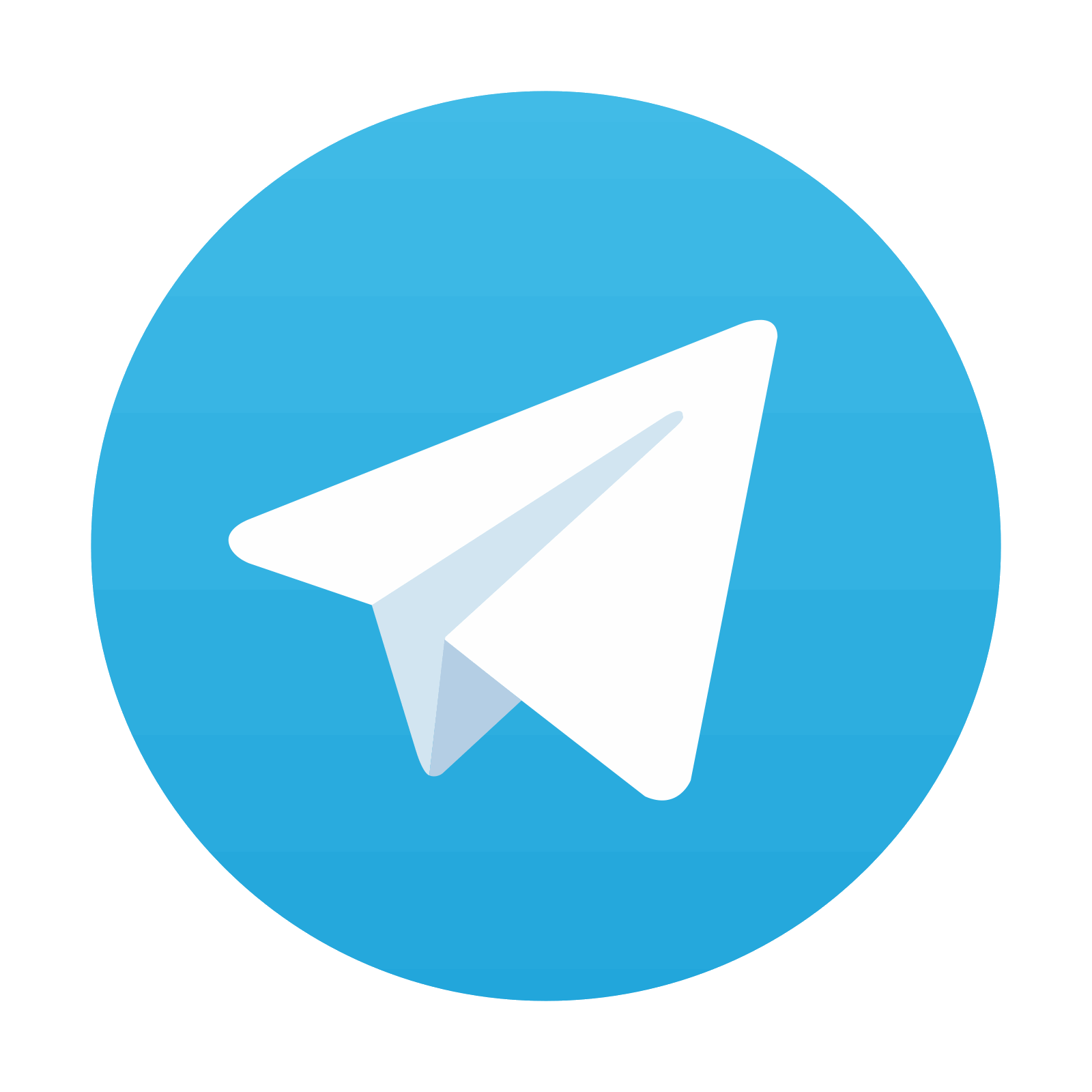
Stay updated, free articles. Join our Telegram channel
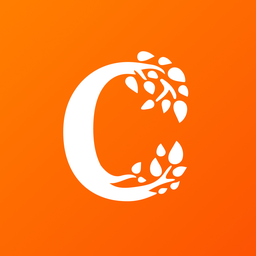
Full access? Get Clinical Tree
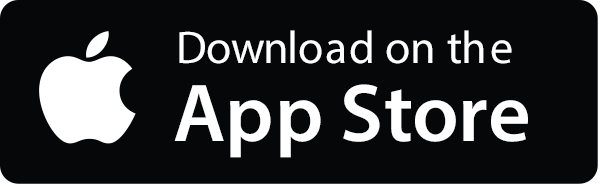
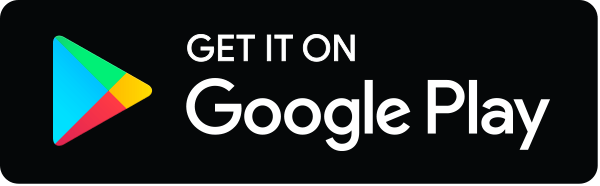