Raymond Y. Kwong
Cardiovascular Magnetic Resonance Imaging
With excellent spatial and temporal resolution, unrestricted tomographic fields, and no exposure to ionizing radiation, cardiac magnetic resonance imaging (CMR) provides morphologic and functional information relevant to a broad array of cardiovascular diseases. This chapter reviews the current evidence for use of CMR in diagnosing and treating cardiovacular disease.
Basic Principles of Magnetic Resonance Imaging
The Magnetic Field and the Gradient Coil System
Magnetic resonance imaging (MRI) is based on imaging of protons within the abundant hydrogen atoms in the human body. The hydrogen protons behave like tiny magnets. When a patient is placed inside the CMR scanner within a static magnetic field (called B0), spins either align with or opposite of the main direction of B0. The summation of the aligned and opposing spins forms a net magnetization vector that aligns along the longitudinal axis (z axis) of the magnet at static state before deposition of any radiofrequency (RF) pulse. B0 is designed to have the same strength along each of the three orthogonal directions (designated x, y, and z) inside the CMR bore; thus it is a homogeneous magnetic field. The homogeneous B0 is fine-tuned by the computer-controlled adjustments of currents in small coils mounted within the magnet (known as active shimming). Apart from lining up with B0, spins also precess (wobble about the axis of the B0 field) at a frequency ω0 (the Larmor frequency) proportional to B0 as described by the following equation: ω0 = γB0, where γ is the gyromagnetic ratio (a constant for hydrogen for a given field strength). In order to introduce a system of spatial address of the Larmor frequency, three orthogonal sets of gradient coils are placed so that a slight linear alteration in the strength of B0 can be created in each of the x, y, and z directions. As a result, magnetic spins precess at frequencies according to their locations along each of the three orthogonal axes, and they can be selectively excited by specific radiofrequency pulses.1
Generation of Magnetic Resonance Signal, Signal Contrast, and Image Formation
In order to create a magnetic resonance image, an RF pulse with a frequency matched to the Larmour frequency of the magnetic spins will excite magnetic spins of interest to a higher energy state, which leads to transition of the net magnetization vector from the z axis onto the x-y plane. The extent to which the magnetization vector is tipped away from the direction of B0 (z axis) defines the flip angle, reflects the amount of energy deposition in tissue, and is a function of the strength and duration of the RF pulse. The magnitude of the vector onto the x-y plane will determine the amount of signal generated, which is received by a set of surface coils. For the purpose of imaging a specific slice plane through the body, the magnetic gradient causes a spread of Larmor frequencies perpendicular to a prescribed slice plane. The RF pulse will then excite only the slice plane with magnetic spins precessing at frequencies matching the frequency bandwidth of the RF pulse.
The absorbed electromagnetic energy will be released by two coexisting mechanisms, longitudinal magnetization recovery and transverse magnetization decay. Longitudinal magnetization recovery corresponds to the exponential rate of recovery of the longitudinal component (z-direction) of the magnetization vector, characterized by a time constant, T1, which is defined as the time to recover 63% of the original longitudinal magnetization vector. T1 is a physical characteristic of tissue and is affected by the field strength of the scanner, with values progressively greater (longer times) at higher field strengths (in Tesla units). T1 characterization therefore allows generation of images that reflect the differences of T1 between tissue types. A T1-weighted scan will keep the time between delivery of two successive flip angles (repetition time) short, so tissues with different T1 values will demonstrate different signal intensity as they follow a T1 recovery. The transverse magnetization decay results from interaction between neighboring spins (spin-spin interaction) leading to exponential loss of the transverse component of the net magnetization vector, defined by the time constant T2. T2 also is a tissue-specific parameter and is defined as the time to lose 63% of the transverse magnetization. Unlike T1 values, T2 values are less related to the field strength of the scanner. The choice of signal contrast weighting of the imaging method is dictated in part by the physiologic characteristics of the tissue being studied. For qualitative interpretation, signal enhancement (from T1 effects) is in general preferred over darkening (T2*) (see explanation further on) effects, so most pulse sequences used in CMR are relative T1-weighted signal-enhancing techniques. T2-weighted and T2*-weighted CMR are primarily used for imaging of myocardial edema and iron content, respectively. With the application of magnetic field gradients in any of the three orthogonal directions, the magnetic resonance signal can carry spatial localization information, produced by encoding steps known as slice select, phase encoding, and frequency encoding. All relevant information of the magnetic resonance signal is stored in a data matrix called the k-space, which will undergo two-dimensional inverse Fourier transformation to form an image.
Patient Safety in Cardiac Magnetic Resonance
Clinical CMR scanners generate strong magnetic fields. The magnetic field component can be disabled with difficulty by evaporating the cooling liquid helium to the outside environment, but this action carries significant risk and is associated with high restoration costs. Common implants hazardous in CMR scanning include cochlear implants, neurostimulators, hydrocephalus shunts, metal-containing ocular implants, pacing wires, and metallic cerebral aneurysm clips. A full list is available at www.mrisafety.com (the official website for the Institute for Magnetic Resonance Safety, Education, and Research). Sternal wires, mechanical heart valves, annuloplasty rings, coronary stents, nonmetallic catheters, and orthopedic or dental implants are safe. Most claustrophobic patients can be managed with oral sedation alone or the use of a scanner with large bore size.
The risks associated with performing MRI in patients with a pacemaker or an implantable cardioverter-defibrillator (ICD) (see Chapter 36) include generation of an electrical current from the metallic hardware, device movement induced by the magnetic field, inappropriate discharging and sensing, and heating as a result of the “antenna effect.” However, a number of experienced centers have reported safety in performing CMR in a controlled setting in patients who have recent pacemaker models and are not pacemaker-dependent. The first pacemaker designed to allow MRI scanning has been approved by the U.S. Food and Drug Administration (FDA), but imaging over the chest and neck region currently is not recommended.
Cardiac Magnetic Resonance Assessment of Specific Disorders and Conditions
Discussed in this section are clinical applications of CMR. Table e17-2 summarizes the CMR protocols, by study indications, used at our center. A detailed description of CMR protocols endorsed by the Society of Cardiovascular Magnetic Resonance (SCMR) can be found at www.scmr.org.7 In addition, the SCMR has established reporting guidelines to provide a framework for enhancing communication with referring physicians.8
TABLE e17-2
Typical Cardiac Magnetic Resonance Protocols at Brigham and Women’s Hospital
STUDY INDICATION(S) | TECHNIQUES OF HIGH RELEVANCE | TYPICAL SCAN PLANES | OPTIONAL TECHNIQUES |
Myocardial viability for benefit from coronary revascularization | Short-axis stack and selected long-axis locations | ||
Myocardial ischemia Vasodilating stress Dobutamine stress | Short-axis stack and selected long-axis locations 3 short-axis and 2 or 3 long-axis locations for stress cine | ||
Acute myocardial infarction | Short-axis stack and selected long-axis locations | ||
Detecting ACS or other causes of myocardial injury | Short-axis stack and selected long-axis locations | ||
Assessing the etiology of an undiagnosed cardiomyopathy or a specific cardiomyopathy | Short-axis stack and selected long-axis or axial locations | ||
Pericardial disease | Short-axis stack and selected long-axis or axial/oblique locations | ||
Valvular heart disease | Short-axis stack and selected long-axis or axial/oblique locations | ||
Cardiac mass/thrombus | Short-axis stack and selected long-axis or axial/oblique locations | ||
CMR for left atrial mapping and pulmonary vein ablation | Short-axis stack and selected long-axis Coronal 3D locations for MRA |
ACS = acute coronary syndrome; CMP = cardiomyopathy; EGE = early gadolinium enhancement; FGRE = fast gradient recalled echo; HCM = hypertrophic obstructive cardiomyopathy; 3D = three-dimensional; T1W = T1-weighted; T2W = T2 weighted.
Coronary Artery Disease
Current CMR protocol for coronary artery disease (CAD) integrates cine imaging, T2-weighted edema imaging, myocardial perfusion at rest and stress, and LGE imaging of MI and provides a comprehensive evaluation of myocardial anatomy and physiology. Coronary MRA is done as a part of the examination in more experienced centers. As noted, Table e17-2 summarizes the CMR protocols used in our center; the typical CMR findings are described in Table e17-3.
Myocardial Infarction
LGE imaging currently is the most accurate noninvasive method for quantifying infarct size and morphology. Infarct size estimated by LGE imaging has been well validated against histologic pattern, and commercial software systems are available to perform infarct size quantitation. With an excellent spatial resolution of 1.5 to 2 mm and a high contrast-to-noise ratio, LGE imaging provides detection of subendocardial infarction beyond either single photon emission computed tomography (SPECT) or positron emission tomography (PET) imaging (see Chapter 16). The robustness of the LGE imaging across MRI models was demonstrated by a double-blinded multicenter randomized clinical trial in which LGE was shown to detect acute and chronic infarcts with a sensitivity of 99% and 94%, respectively.9 In the acute MI setting, when LGE imaging is performed early (within the first 5 minutes) after contrast injection, microvascular obstruction (no-reflow) can be seen as a dense hypoenhanced area surrounded by a bright region representing the infarct (Fig. 17-7). This noninvasive method for quantifying microvascular obstruction has been validated against angiographic parameters of microcirculatory flow. Recent reports have even demonstrated detection of myocardial hemorrhage as a result of reperfusion injury (Fig. 17-8; Video 17-5). Acute right ventricular (RV) injury also can be detected at high sensitivity (see Fig. 17-7). Some evidence suggests that the high spatial resolution and contrast-to-noise ratio of LGE imaging translate to useful patient prognostic information. For patients with acute MI, presence of either microvascular obstruction or acute RV injury has prognostic implication independent of left ventricular (LV) infarct size and LV ejection fraction (LVEF).10 In the nonacute setting, an infarct identified solely by LGE imaging in patients without a history or ECG evidence of MI or in patients with diabetes, is a strong predictor of adverse events, independent of common clinical risk markers.11 The strength of LGE imaging in detecting clinically unrecognized MI has been extended to the population level. A recent large community-based cohort study demonstrated that LGE imaging detected a very high prevalence of unrecognized (and hence untreated) MI in older persons, which was missed by ECG. This group of patients experienced remarkably increased mortality risk.12
Several pilot studies demonstrated that infarct tissue heterogeneity quantified from LGE images may describe arrhythmogenic substrates that develop as the result of an MI. Schmidt and co-workers reported that monomorphic ventricular tachycardia during electrophysiologic studies was more strongly associated with infarct heterogeneity than with LVEF.13 Roes and colleagues found that infarct heterogeneity was a strong predictor of spontaneous ventricular arrhythmias necessitating appropriate ICD therapy in patients with MI.14 These findings are concordant with the observed association between infarct tissue heterogeneity and patient mortality in yet another study.15
Assessment of Myocardial Viability and Benefit from Coronary Revascularization
CMR allows multifaceted assessment of structure and physiology associated with myocardial viability. End-diastolic wall thickness alone has limited accuracy in predicting recovery of segmental function because the wall tissue may include irreversibly damaged myocardium and a thinned epicardial rim of viable myocardium. From early cine CMR studies, it has been shown that end-diastolic wall thickness of 5.5 mm or greater and dobutamine-induced systolic wall thickening of 2 mm or greater have excellent specificity and sensitivity in the prediction of segmental contractile recovery after revascularization (sensitivity, 89%; specificity, 94%). In a landmark paper by Kim and colleagues, the transmural extent of myocardial scar detected by LGE imaging was shown to accurately predict a progressive stepwise decrease in functional recovery despite successful coronary revascularization.16 This prediction of segmental functional recovery was especially strong in segments with resting akinesia or dyskinesia. Compared with dobutamine cine CMR, LGE imaging is easy to perform and interpret, and a 50% transmurality cut-off point is sensitive in predicting segmental contractile recovery. Even very thin myocardial regions without LGE have the potential for increase in thickness and recovery of function after revascularization.17 On the other hand, the high specificity from low-dose dobutamine cine imaging provides a physiologic assessment of the midmyocardial and subepicardial contractile reserve, particularly in segments with subendocardial MI involving less than 50% of the transmural extent. At our center, it appears that LGE imaging alone suffices for answering most questions raised in imaging for myocardial viability. However, low-dose dobutamine cine CMR can be complementary in assessing myocardial viability early after acute MI when tissue edema is prominent or when high test specificity is demanded to justify bypass surgery in patients at high preoperative risk.
Many earlier imaging-based viability studies were limited by retrospective design, lack of treatment assignment, and use of recovery of segmental function as an endpoint that provides little information about long-term patient outcomes. The Surgical Treatment of Ischemic Heart Failure (STICH) trial overcame the limitations of previous studies by prospectively assessing the role of viability imaging in decision-making toward cardiac bypass surgery or aggressive medical therapy in patients with CAD and LVEF below 35%.18 Although detection of myocardial viability was associated with patient survival, SPECT perfusion imaging or dobutamine echocardiography failed to identify patients who will derive the greatest survival benefit from addition of coronary artery bypass grafting (CABG) to aggressive medical therapy. One can postulate that because CMR can interrogate multiple targets of myocardial viability, it may provide a more precise viability assessment than SPECT or dobutamine echocardiography and may be more useful in guiding decision making in patients such as those studied in the STICH trial. Prospective studies of this issue are needed.
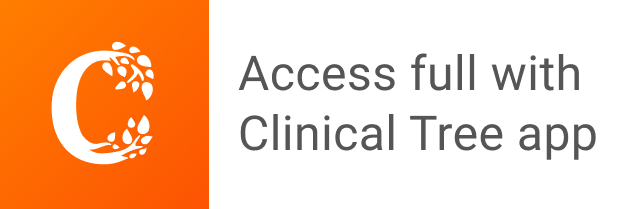