Cardiac Ion Channels
Cardiac myocytes are activated by an electrical signal, the action potential, in which changes in electrical potential across the plasma membrane (Em) result from an elaborate sequence of openings and closing of ion channels. These action potentials, which differ in various regions of the heart, organize the mechanical events of the cardiac cycle and help provide the homogeneity of activation needed to optimize the efficiency of cardiac contraction (see Chapter 6).
Membrane Potential
A microelectrode inserted through the plasma membrane of a resting cardiac myocyte records an intracellular potential that is more negative than that outside the cell (Fig. 13-1). How this potential difference is described is determined by convention. When expressed in terms of the potential recorded within the cell, with the surrounding medium viewed as zero, resting potential is negative. If, on the other hand, resting potential is described in terms of the potential outside the cell, and intracellular potential viewed as zero, resting potential is positive.
Much confusion arises because different conventions are used in cardiac electrophysiology and electrocardiography! Membrane electrophysiologists, who place electrodes inside the cell and measure the potential of the cell interior relative to that in the surrounding medium, describe resting potential as negative, whereas clinical electrocardiographers, who place electrodes on the body surface, view the myocardium from the outside, and describe resting potential as positive. This chapter and Chapter 14, which describe cellular events, view resting potential as negative, whereas Chapters 15 and 16, which describe electrocardiograms, view resting cells as positively charged.
A change in membrane potential that decreases the electronegativity inside a resting myocyte is called depolarization (Fig. 13-2). The return of membrane potential toward its resting level in a depolarized myocyte is repolarization, whereas an increase in the resting potential of an unexcited cell is hyperpolarization. Action potentials must include at least two phases: one of depolarization; the other of repolarization. Action potential amplitude, which is usually expressed in millivolts (mV), defines the extent to which cellular electronegativity changes from its resting level, including any reversal to electropositivity.
Membrane Currents
The most important physiological currents in the heart are carried by ions that cross the plasma membrane, and so are ionic currents. By convention, these are described as if they were carried by positively charged ions. Capacitive currents, on the other hand, are generated by electron fluxes toward and away from the surfaces of the plasma membrane.
![]() Fig. 13-1: Resting cardiac muscle cell. The normal resting potential is negative inside the branched myocytes and positive in the extracellular space. |
An inward current, according to electrophysiological convention, is the flux of charge that would occur if a positive ion moved across the plasma membrane into the cell. Because the interior of the resting cell is negatively charged, inward currents cause depolarization. Most inward currents in the heart are generated when positively charged sodium and calcium ions enter the cell; however, the outward movement of a negative ion has the same effect on membrane potential as the inward movement of a positive ion, so that the efflux of an anion like chloride also generates an inward current (Table 13-1). An outward current can be generated when a positively charged ion leaves the cell interior, or when a negatively charged ion enters the cell. Both increase electronegativity within the cell. In resting cells, outward currents cause hyperpolarization, while outward currents that follow depolarization, and so return membrane potential toward the resting negativity, cause repolarization (Fig. 13-2).
Unlike ionic currents, which are generated when ions cross the lipid bilayer through pores in the plasma membrane, capacitive currents are generated by the movements of electrons relative to the membrane surface (Fig. 13-3). The latter occur in biological tissues because the phospholipid bilayer is a capacitor which is made up of an insulator (the hydrophobic core) that is surrounded by two layers of polar molecules (the phospholipid head groups) (see Chapter 1). A cathode placed outside a resting cell, where the plasma membrane is positively charged, reduces the positive charge on the extracellular surface of the membrane and, at the same time, causes
negative charge to move away from the intracellular surface. These charge movements, which are carried by electrons, cause the membrane to depolarize by discharging membrane capacitance.
negative charge to move away from the intracellular surface. These charge movements, which are carried by electrons, cause the membrane to depolarize by discharging membrane capacitance.
Table 13-1 Ions as Charge Carriers Across Cell Membranes | |||||||||||||||||||||||||
---|---|---|---|---|---|---|---|---|---|---|---|---|---|---|---|---|---|---|---|---|---|---|---|---|---|
|
Action potentials are normally initiated by capacitive currents that are generated when a wave of depolarization approaches a region that contains resting cardiac myocytes. These currents depolarize the resting membrane when the approaching wave of depolarization causes the extracellular surface of the plasma membrane to become negative and the interior surface to become positive. Action potentials are propagated along the cell surface when depolarization opens voltage-gated ion channels that carry additional inward current, which cause this process to become regenerative (see Chapter 14).
Membrane Resistance, Permeability, and Conductance
The ratio between membrane potential and current flow is membrane resistance, which can be described by Ohm’s law:

where R is resistance, E is potential, and I is current flow. Membrane conductance, designated g, is the reciprocal of membrane resistance, so that

Permeability and conductance both define the ability of a substance to cross a membrane, but they are not the same. Permeability (P) is the ability of a membrane to allow the movement (flux) of molecules in both directions, from either side of the membrane to the other, whereas conductance describes the movement of charge in one direction across the membrane.
Permeability, which is commonly used to describe systems that are at or near equilibrium, is defined by the relationship:

which states that the flux of a molecule is equal to the permeability coefficient for the molecule (-Pmolecule) times the difference in the concentration of the molecule at the two sides of the membrane (δcmolecule).
Conductance, which characterizes the electric current generated by the movement of an ion across a membrane down a pre-existing electrochemical gradient, is defined by the relationship:

which states that the current generated by an ion flux (iion) is determined by the conductance of the membrane for the ion (gion) and the electromotive force that drives the ion across the membrane (Em – Eion), which is the difference between the actual transmembrane potential (Em) and the transmembrane potential at which there would be no net ion flux (Eion). The latter is called the equilibrium potential for the ion (see Chapter 14). For example, the current carried by potassium ions (iK) is:

where gK is the potassium conductance and (Em – EK) is the electromotive force that moves potassium ions across the membrane. Depending on the difference between Em and EK, current can flow in either direction across the membrane, although not in both directions at any given time. Biological currents are expressed in Siemens, a unit that has the same units as mho, the reciprocal of the ohm that measures resistance.
Changing membrane potential can modify the conductance of a voltage-gated ion channel for a given ion. This property, called rectification, plays an important role in regulating changes in the potential across biological membranes (see Chapter 14).
Generation of the Action Potential
In 1902, Bernstein, who knew that mammalian cells contain a high concentration of potassium, proposed that the plasma membrane is selectively permeable to potassium ions and that fixed negative charges on the cytosolic proteins establish a Donnan equilibrium which concentrates potassium ions in an electronegative cytosol (Bernstein, 1902). This hypothesis was confirmed shortly before the outbreak of World War II, when intracellular potentials were first measured by Hodgkin and Huxley (1945) in England, and Cole and Curtis (1941) in the United States, who inserted microelectrodes into squid giant axons. Their initial measurements confirmed Bernstein’s prediction that the interior of resting cells was electronegative, and that this negativity decreased during excitation. However, intracellular recordings carried out after World War II, when more advanced equipment had become available, yielded a surprise. This was that membrane potential did not simply decrease to zero during excitation, as would be expected if reduction of potassium permeability
alone was responsible for activation. Instead, membrane potential was found to reverse during excitation, when the cell interior became positive. This meant that action potentials are generated by processes more complex than dissipation of the potassium gradient.
alone was responsible for activation. Instead, membrane potential was found to reverse during excitation, when the cell interior became positive. This meant that action potentials are generated by processes more complex than dissipation of the potassium gradient.
The Voltage Clamp
In the 1950s, Hodgkin, Huxley, and B. Katz published a series of classical experiments that characterized the ionic basis for the action potential in squid giant axons. Conceptually, their approach was simple: Instead of measuring potential changes during an action potential, when ionic currents depolarized and repolarized the membrane, they measured the currents needed to hold membrane potential at a constant level. This was accomplished by placing an electrode inside the axon, exciting the membrane, and then applying currents across the membrane to keep membrane potential from changing. The applied currents, which exactly matched the currents that would have otherwise caused membrane potential to change, represent the “voltage clamp.” Measurements of these applied currents made it possible to quantify both the magnitude and timing of physiological ionic currents that, by flowing in the opposite direction, generated the squid axon action potential. Interventions such as changing sodium and potassium concentrations inside and outside the axon demonstrated that depolarization occurs when sodium ions enter the cell, that repolarization is caused by potassium efflux, and that reversal of membrane potential at the peak of the action potential is due to the large sodium influx.
General Properties of Plasma Membrane Ionic Currents
Most of the ion fluxes responsible for plasma membrane depolarization and repolarization do not require the expenditure of energy because the ions move downhill along their electrochemical gradients. These ion fluxes are mediated by members of an extended family of membrane proteins that contain ion-selective pores which, when open, favor the passage of a single ion species. In most cardiac channel proteins, changes in membrane potential open and close these pores, so that these ion channels are generally referred to as voltage-gated.
Most voltage-gated ion channels, once opened, do not remain in the open state, but instead cycle through at least two closed states (Fig. 13-4). The physiological transition of the heart’s sodium and calcium channels from the closed (resting) to the open state, called activation, occurs when depolarization increases the probability of channel opening (see below). At the same time, however, depolarization also increases the probability that these channels will assume the closed (inactive) state; a process that is called inactivation. This allows the depolarizing signal that initially opens a resting channel to cause its subsequent closure. Once closed, the channel assumes a refractory state, called the closed (inactive) state, where the channel cannot be reopened by additional depolarizing stimuli.
A single depolarization causes sodium and calcium channels to open transiently because activation is faster than inactivation. This dual response to membrane depolarization is an example of a general principle in biological regulation, discussed in Chapter 8, that mechanisms which initiate a response also prevent runaway signaling by initiating slower processes that end the response. In this way, ion channels resemble the water taps commonly found in public washrooms, where flooding is prevented when the same signal that starts the flow of water also shuts off the tap, albeit more slowly.
Reactivation (recovery), the transition from the closed (inactive) state to the closed (resting) state where the channel can again be opened by depolarization, requires an additional signal. In the heart, sodium and calcium channels are reactivated when membrane potential is returned to its resting level by repolarizing (outward) currents carried by potassium channels. Once sodium and calcium channels are reactivated and their refractoriness ends, they can again be opened by depolarizing stimuli.
Ion Channel Gating
The mechanisms that control the transitions between the various states of an ion channel are often called gating mechanisms. Initially presented as coefficients in mathematical equations that describe the opening and closing of the channels in the squid axon (Hodgkin and Huxley, 1952), channel gating mechanisms can now be related to conformational changes in specific regions of ion channel proteins.
The equations developed to characterize the behavior of the sodium current that depolarizes the squid axon contain two coefficients, m and h, that characterize the regulation of channel conductance. Because m is a coefficient of channel opening while h is a coefficient of channel closing, sodium current is maximal when m is 1 (100% probability of being open) and h is 1 (0% probability of being closed). This relationship is described by the following modification of Equation 13-4:

Table 13-2 Sodium Channel Gating | ||||||||||||
---|---|---|---|---|---|---|---|---|---|---|---|---|
|
Equation 13-6 states that the inward sodium current (iNa) is determined by the maximal sodium conductance (gNa), m and h, and the difference between actual membrane potential (Em) and the sodium equilibrium potential (ENa). The latter, which is generally about +20 mV, is the potential that would be recorded if the membrane were permeable only to sodium ions (see Chapter 14). The coefficients m and h are now known to describe the properties of two regions of the sodium channel, called the “m gate” and “h gate” (Fig. 13-2). The fact that m appears in the third power initially suggested that each channel contains three m gates; the correct number, as described below, is now known to be four.
Hodgkin and Huxley predicted that depolarization of the resting membrane rapidly opens the m gate while, at the same time, causing the h gate to close more slowly. These different time-dependent properties of m and h explain why the first effect of depolarization is to activate the channel by rapidly opening the m gates, why slower closure of the h gate inactivates the channel, and why the open state is brief. The Hodgkin–Huxley equations therefore define three channel states: an open state in which both gates are open, and two closed states (Table 13-2). In one of the latter, the m gate is closed and the h gate open (closed, resting); in the other, the m gate is open and the h gate is closed (closed, inactive). The difference between the two closed states is that in the closed, resting state, the m gate can be opened by membrane depolarization, while in the closed, inactive state, the h gate can be opened only by repolarizing potassium currents that return membrane potential to its resting level (Fig. 13-4). Because the m gates remain closed during reactivation, sodium channels do not reopen unless the membrane is depolarized again.
Hodgkin and Huxley also developed equations to describe the squid axon potassium channel, whose opening was found to depend on the fourth power of a coefficient that they called n:

One of the more remarkable facts about the Hodgkin–Huxley equations is that they anticipated key features of the structure of voltage-gated ion channels. For example, each sodium channel contains four α-helical transmembrane segment rich in positively charged amino acids that are now known to be the activation (m) gates, while the inactivation (h) gate is a single intracellular peptide chain that responds to depolarization by assuming a conformation that occludes the inner mouth of the channel pore (see below).
Ion Channel Proteins
Voltage-gated ion channels are members of a family of tetrameric proteins that evolved from a monomeric ancestor that, through gene duplication and divergence, gave rise to the modern channels (Fig. 13-5). Around the time that eukaryotes evolved from prokaryotes, the ancestral
monomeric channel protein gave rise to hyperpolarization-activated channels and potassium channels whose four subunits are not linked covalently. Calcium channels are the most primitive voltage-gated channels whose four subunits are covalently linked; later, when multicellular metazoan phyla evolved at the beginning of the Cambrian period, the gene encoding the calcium channels diverged to give rise to sodium channels, whose large action potentials conduct much more rapidly than calcium-dependent action potentials. The rapid conduction made possible by these sodium currents (see Chapter 16) is essential for allowing multicellular organisms to coordinate the movements of the different regions of their bodies.
monomeric channel protein gave rise to hyperpolarization-activated channels and potassium channels whose four subunits are not linked covalently. Calcium channels are the most primitive voltage-gated channels whose four subunits are covalently linked; later, when multicellular metazoan phyla evolved at the beginning of the Cambrian period, the gene encoding the calcium channels diverged to give rise to sodium channels, whose large action potentials conduct much more rapidly than calcium-dependent action potentials. The rapid conduction made possible by these sodium currents (see Chapter 16) is essential for allowing multicellular organisms to coordinate the movements of the different regions of their bodies.
![]() Fig. 13-5: Molecular architecture of a potassium channel as represented in “Birth of an Idea,” a sculpture by Julian Voss-Andrae in 2007 that is based on atomic coordinates determined by Zhou et al. (2001). The sculpture shows the pore, depicted as contained within a wire mesh, and several surrounding transmembrane α-helices. (Commissioned by Roderick MacKinnon and reproduced under the terms of the GNU Free Documentation License). |
Pores and Gates
One of the fundamental properties of plasma membrane channels is their selectivity, which allows calcium channels to conduct calcium ions, sodium channels to conduct sodium ions, etc. Although this selectivity is not always absolute, the preferences of many channels for a given ion can be very stringent, as in calcium channels, which under physiological conditions conduct mainly calcium in spite of the almost hundredfold greater concentration of sodium ions in the extracellular fluid. Selectivity is made possible by ion-binding sites within the channel that allow only the “correct” ion to enter the pore. For example, the anionic groups of potassium channels are arranged within the pore in a conformation that recognizes potassium ions (Fig. 13-6). Because other ion species bind with lower affinity to these anionic sites, they are excluded from the selectivity filter by the bound potassium ions. The energy for the ion flux is provided by the concentration gradient across the bilayer; ions enter the channel from the side of the membrane containing the higher ion concentration because these ions are most likely to displace ions already bound within the channel.
Gating Currents, Inactivation Gates, and Inactivation Particles
To explain the ability of changing membrane potential to open and close voltage-gated ion channels, Hodgkin and Huxley predicted that the gating regions contain charged regions that move in response to changing membrane potential. This led to the prediction that movement of these charged regions in response to changing membrane potential would generate small “gating currents.” In the 1960s, careful measurements carried out under conditions that eliminated or corrected for changing membrane capacitance and ion fluxes were able to identify the gating currents caused by movements of positively charged regions of the sodium channel that correspond to the activation (m) gates (see below).
Closure of the h gates is now known to occur when depolarization causes a conformational change in an intracellular region of the sodium channel. This leads to the formation of an “inactivation particle” within the cytosol that blocks the inner pore of the open channel (Fig. 13-7).
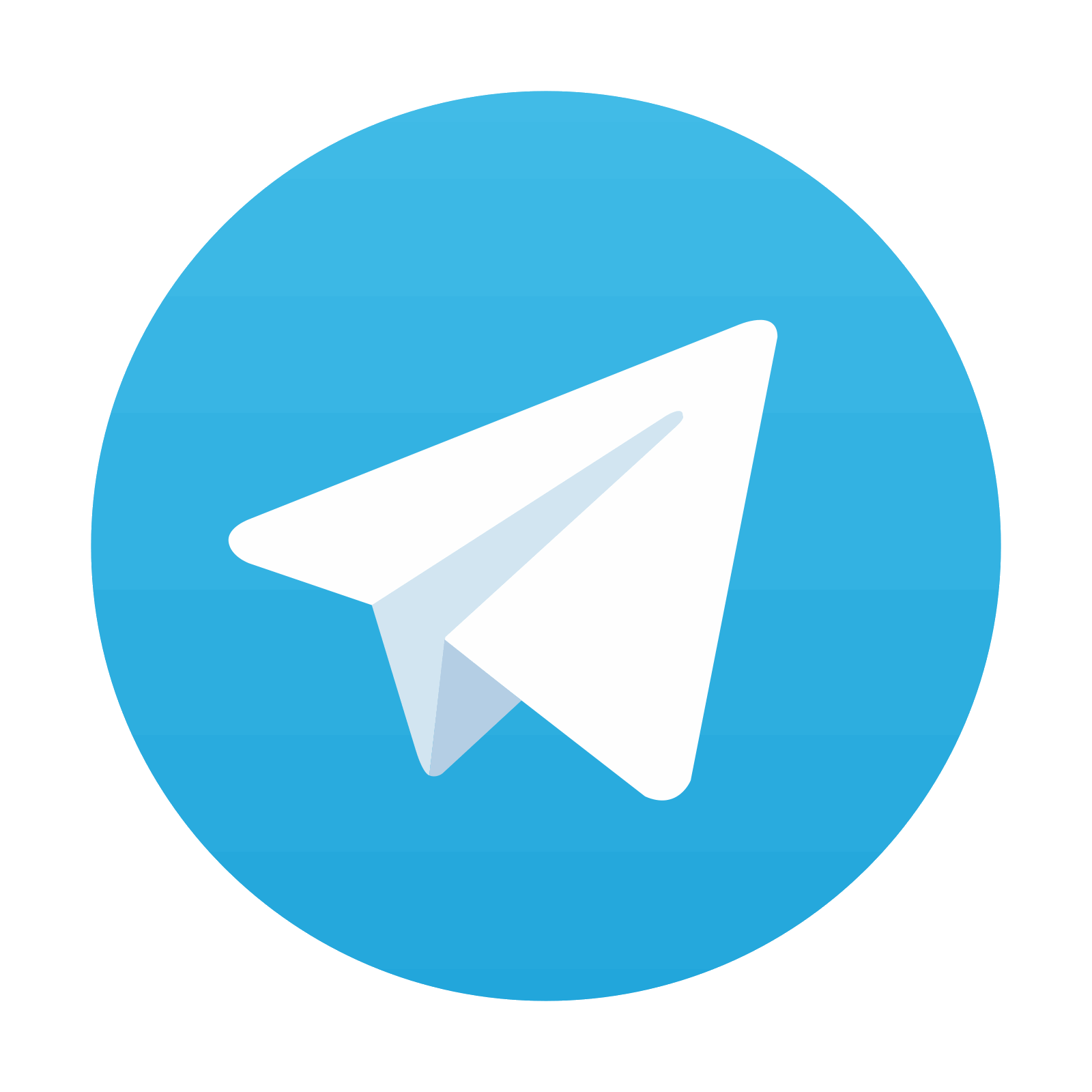
Stay updated, free articles. Join our Telegram channel
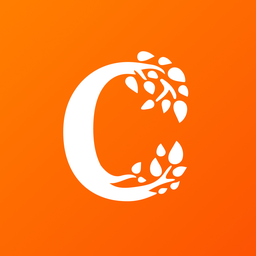
Full access? Get Clinical Tree
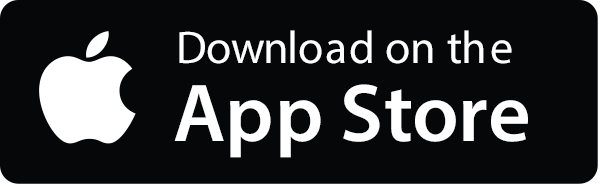
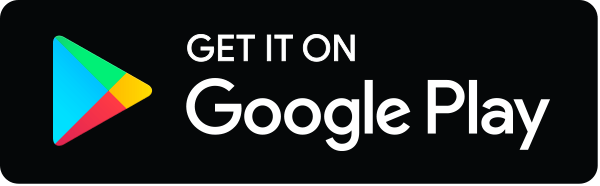