Cardiac Catheterization and Angiography
Nathaniel W. Taggart
Allison K. Cabalka
Cardiac catheterization has a long and illustrious history, beginning in 1929 when Werner Forssmann (1), a surgical resident and future urologist, performed the first cardiac catheterization from an arm vein—on himself. He was subsequently awarded the Nobel Prize for his discovery. In the 1950s, the catheterization laboratory was used to understand the physiology of congenital heart defects. By the 1960s to 1970s, advances in cardiac surgery required more detailed anatomic information, which was addressed using axial angiography (2,3). In the 1980s, 2-D echocardiography made it possible for many patients to be diagnosed and treated without cardiac catheterization. In the 1990s, transesophageal echocardiography, computerized tomography, and magnetic resonance imaging were used to produce detailed cardiac images, further decreasing the need for diagnostic cardiac catheterization. However, as more complex cardiac conditions are treated, more detailed physiologic data are necessary for the evaluation and treatment of children with congenital or acquired heart defects. This chapter discusses the acquisition of hemodynamic data and angiographic images. Interventional techniques are discussed separately in Chapter 17.
Diagnostic Cardiac Catheterization and Angiography
Indications
A thorough diagnostic cardiac catheterization provides complete physiologic and anatomic data. With the appropriate team, the risk of cardiac catheterization is low—usually less than the risk associated with clinical decisions based on inadequate information. The three major indications for performing a diagnostic cardiac catheterization are as follows:
A complete anatomic diagnosis or necessary hemodynamic information cannot be obtained by noninvasive methods.
Clinical signs and symptoms are not consistent with a patient’s diagnosis.
A patient’s clinical course is not progressing as expected.
Indications for catheterization in specific lesions are covered subsequently in the relevant chapters pertaining to each lesion.
Techniques
Planning the Study
In order to acquire complete and accurate information from cardiac catheterization, the cardiologist must have a clear understanding of the specific question(s) to be answered. Necessary preparation before cardiac catheterization includes a thorough review of the patient’s complete history and physical examination, including all previous pertinent noninvasive studies, cardiac catheterizations, and operative notes from all prior surgeries. Additional laboratory studies should be obtained as indicated by the clinical findings including electrolytes in patients taking diuretics, blood urea nitrogen, and creatinine if there is concern for renal insufficiency, pregnancy tests in adolescent and adult females, and blood typing for any patient in whom the complication risk is significant or in whom intervention potentially may be needed. Patients referred for cardiac catheterization may be severely ill or have various comorbidities. Recognition of these coexisting conditions and appropriate anticipation of potential complications is vitally important. Precatheterization planning should incorporate discussion of the case with the anesthesiologist or provider who will be managing patient sedation. Airway management and the use of conscious sedation versus general anesthesia should be discussed and planned in advance of the catheterization procedure.
If a patient is anemic, transfusion prior to cardiac catheterization can optimize the baseline hemodynamic condition. Cyanotic patients who have significantly elevated hemoglobin levels are at increased risk of stroke during cardiac catheterization, due to polycythemia. Partial exchange transfusion may be considered prior to catheterization, but currently is rarely performed.
Premedication, Sedation, and Anesthesia
Physicians and institutions vary in their approaches to premedication, sedation, and anesthesia. Management is influenced by the diversity of cardiac defects and the expertise of the cardiologist and anesthesiology team. The goals of premedication and sedation or anesthesia are to decrease anxiety, facilitate parental separation, ensure comfort, promote amnesia, and facilitate the safe and efficient performance of the procedure. At the same time, sedation and mechanical ventilation may affect intracardiac hemodynamic measurements and can influence the applicability of the data acquired during the catheterization. The level of sedation during the catheterization and the use of supplemental oxygen should be discussed explicitly with the anesthesiologist before the beginning of the procedure. It is now standard to assign a person other than the primary cath physician to be responsible for sedation and airway monitoring. In most cases, this should be a cardiac anesthesiologist, particularly for patients with complex congenital heart disease, elevated pulmonary vascular resistance, or depressed myocardial function.
Prior to the catheterization, in general, pediatric patients should not have solid food for 8 hours, but may have milk or infant formula up to 6 hours prior, breast milk up to 4 hours before, with clear liquids up to 2 hours before the procedure. Certain patients should have an intravenous (IV) line placed in (preferably in an upper extremity) before arriving in the cath lab; this facilitates administering IV fluid (to ensure hydration) and sedation, especially in cyanotic or polycythemic patients. Premedications can be administered orally or intravenously. Some children are best treated with mask induction first, prior to IV placement. The periprocedural management should be individualized for each patient based upon his or her age, level of anxiety, and specific cardiac defect/physiology.
Local anesthesia is administered prior to starting percutaneous access. Lidocaine is the usual anesthetic, which is painful when initially injected. Premedication diminishes the discomfort to lidocaine infiltration, as does buffering the lidocaine with sodium bicarbonate, pretreatment with a topical anesthetic cream (lidocaine 2.5% and prilocaine 2.5%), use of a small needle (25 gauge) to infiltrate the skin prior to infiltrating deeper tissues, and a slow rate of lidocaine infiltration. Care should be taken to assure that lidocaine is not injected intravascularly. The dose of lidocaine should not exceed 6 mg/kg, as an excessive dose or accidental IV administration can cause seizures.
Effective sedation and analgesia can be maintained during the procedure using various IV medications, including fentanyl, midazolam, ketamine, and propofol. Cardiac depressant agents (e.g., propofol) must be used cautiously or avoided in patients with already depressed cardiac function. Vasodilator agents should typically be avoided in patients with tetralogy of Fallot or similar physiology due to severe pulmonary outflow obstruction where systemic vasodilation may produce increasing right-to-left shunt. Conversely, ketamine can increase the systemic vascular resistance and may be useful in certain clinical settings. When administering sedative medications, it is important to observe any changes in heart rate, blood pressure, and pulse oximetry and to have appropriate airway support immediately available. In the current era, most congenital cardiac catheterization procedures in pediatric patients are performed with general anesthesia, so the involvement of a cardiac anesthesiologist is paramount to assure a safe procedure.
Vascular Access
Establishing reliable vascular access is a vital early step to conducting a safe and efficient cardiac catheterization. Particularly in young children and neonates, hurried attempts at vascular access can result in significant bleeding or vessel damage, which in turn makes access more difficult.
Vascular access is most commonly obtained in the femoral system. From this approach, right heart catheterization is performed in an antegrade fashion through the great veins. Left heart catheterization is often performed in a retrograde fashion via the femoral artery, but can also be accomplished antegrade across an atrial septal defect, patent foramen ovale (PFO), or via transseptal puncture. The type and location of vascular access necessary to perform the study are dictated both by the patient’s particular anatomy and the objectives of the catheterization. For example, patients with a bidirectional cavopulmonary superior vena cava (SVC) to pulmonary artery anastomosis without interruption of the inferior vena cava require internal jugular (IJ) or subclavian vein access to enter the pulmonary arterial tree.
Patients with congenital heart disease who have undergone multiple prior catheterizations and surgical operations may have obstruction of the systemic veins or femoral arteries, complicating vascular access. Previous catheterization reports can provide insight into difficulty with vascular access and whether complete vessel occlusion has been documented. Certainly, it is helpful to future operators if this is documented in the medical record, if not also with a digital record of the hand injection of contrast in the femoral venous system for confirmation. In such cases, alternative sites of vascular access should be explored. Occasionally, vascular ultrasound with color flow Doppler or other imaging modalities may be necessary prior to catheterization to identify available sites. The most common sites of venous access for cardiac catheterization are femoral, IJ, and subclavian. Percutaneous arterial access is almost exclusively achieved via the femoral artery, although radial artery access is becoming an alternative approach in larger children and adults. Other modes of venous access include use of the umbilical vein or artery in the newborn or transhepatic route in patients who have multiple obstructed systemic veins.
Femoral Approach
Atraumatic percutaneous entry of the femoral vessels should be possible in nearly all pediatric patients. This requires knowledge of the anatomy, attention to detail, excellent technique, and patience. The most common approach, using a plain-beveled needle with Seldinger technique, is described here (4).
The patient is positioned on the catheterization table with the hips elevated slightly, and the legs restrained in a straight or slightly inward rotation. Conversely, some physicians prefer to have the legs rotated outward in a more “frog-legged” position; this displaces the femoral vessels to a more lateral position. The anatomic landmarks are identified both before and after draping the patient. Consistent landmarks for determining the site of vessel entry are the anterior superior iliac spine, the symphysis pubis, the inguinal ligament, and the femoral pulse. In small patients, the inguinal ligament is palpable. The femoral vessels should be entered 1 to 2 cm below the inguinal ligament to ensure reliable hemostasis at the completion of the procedure. Vessel entry above the inguinal ligament is likely to result in a labial or scrotal hematoma or the more significant complication of retroperitoneal bleeding.
After the skin has been cleansed and a sterile field established, bony and soft tissue landmarks are again palpated to locate the femoral neurovascular bundle. This bundle courses vertically in the upper thigh and passes below the inguinal ligament approximately midway between the symphysis pubis and the anterior superior iliac spine. The femoral artery lies lateral to the vein and medial to the femoral nerve. Palpating the femoral pulse provides a reliable landmark for infiltration of anesthetic and, ultimately, needle puncture of the vein or artery. Ideally, the right femoral vein is accessed, as it provides a straight course to the right atrium (except in patients with situs inversus). Ultrasound with color flow Doppler can help determine vessel patency prior to attempted needle puncture. In the event that vessel patency cannot be documented by ultrasound, attention should be turned to the left-sided femoral vessels, which should also be evaluated by ultrasound. Due to the risk of complications, the use of ultrasound guidance for femoral access is becoming more commonplace (5) except in very young infants in whom the ultrasound probe may compress the vessels.
Once the vessel and surrounding landmarks have been identified, the overlying skin is infiltrated with a small amount of lidocaine, administered gradually to minimize the pain associated with administration. Too large a volume of lidocaine, particularly in small children, can distort the underlying vessels and make access more difficult. Once the skin is appropriately numbed, a hollow-bore needle, preferably with a short bevel, is introduced at a 30- to 45-degree angle to the skin, 1 to 2 cm below the inguinal ligament. The needle should be inserted using an “advance and wait” technique, observing for backflow of blood between 1- and 2-mm advances. If no backflow of blood occurs, once the needle is advanced to the bone, it should be withdrawn slowly and blood return monitored. It is also possible to advance the needle slowly with application of gentle aspiration using a syringe at this point if one is not used initially. Once consistent blood return is obtained, the needle is stabilized, and the soft end of the guidewire is advanced through the needle into the vessel lumen. The wire should advance freely; any resistance should be investigated. When accessing the femoral vein, the wire position can be confirmed with brief fluoroscopic imaging, typically advancing the wire into the distal IVC. When accessing the femoral artery, it should be advanced to the lower abdominal aorta. Once the wire position has been confirmed, the needle is removed and an appropriate-sized hemostatic sheath is placed over the wire and advanced until the sheath hub is at the skin. The sheath is cleared of air by drawing back on a syringe attached to the sheath. Prompt blood return again confirms the position of the sheath within the vessel space, and connection to the monitoring pressure transducer confirms venous or arterial pressure.
When advancing the introducer wire through the needle, if the wire meets resistance immediately upon leaving the needle tip, it is most likely in the extravascular space and should be removed. If there is resistance to removing the wire, then the entire system should be removed to be sure that the wire is not sheared off beyond the end of the needle bevel. However, if the wire extends well beyond the tip of the needle before meeting resistance, the vessel may be obstructed. Obstruction can be confirmed by a small injection of contrast through the needle, using a slip-tip syringe, and imaged under fluoroscopy. This image of the obstructed vessel should be recorded and documented in the catheterization report for future reference.
Internal Jugular Approach
The IJ vein is the most common approach used when femoral veins are obstructed. It is the preferred approach for right ventricular endomyocardial biopsies (except in very young patients). The IJ vein is entered outside the thorax, so there is a low risk of pneumothorax. For appropriate positioning, the patient’s head must remain turned to one side; thus in a sedated patient (particularly an infant), the airway may be compromised. Therefore, general anesthesia with endotracheal intubation is commonly used for IJ access in smaller patients. The right IJ vein is preferred (to the left) because it offers a more direct course into the right atrium, and the apex of the lung is lower on the right side. The patient is positioned with a soft towel roll under the neck to slightly hyperextend it with the head turned to the left. Landmarks to be identified before and after draping the patient are the carotid pulse and the two divisions of the sternocleidomastoid muscle. The right IJ vein lies below the sternocleidomastoid muscle, anterior and lateral to the carotid artery. Ultrasound guidance is mandatory for IJ access, and the IJ vein is visualized just lateral to the carotid artery. This spatial relationship is not universal, however. A growing body of evidence mandates that ultrasound guidance be used during puncture of the IJ vein to improve access success and decrease vascular complications (6,7,8,9). The IJ vein is distinguished from the carotid artery as gentle pressure with the transducer compresses the vein, and the artery has obvious pulsations with the heart rate. The skin is infiltrated with a small amount of lidocaine. With simultaneous ultrasound guidance, the access needle is advanced with constant gentle aspiration on the syringe until a free flow of blood return is obtained. Using fluoroscopic guidance, a guidewire is passed through the needle, into the vein, and is then advanced into the right atrium (or pulmonary artery in patients with a cavopulmonary anastomosis).
Subclavian Approach
The subclavian vein is posterior to the clavicle, superficial and inferior to the subclavian artery, lying partly on the pleura. As a result, complications of subclavian vein access include pneumothorax, hemothorax, and intravascular air. Complications are more likely to occur when there is pulmonary parenchymal disease, pulmonary hypertension, or anatomic thoracic abnormalities (including previous surgery).
The patient is positioned with a small roll under the shoulders. Airway adequacy is verified before proceeding. Prior to draping the patient and again before vessel entry, one should identify important landmarks, specifically the suprasternal notch and the depression at the lateral third of the clavicle. With the usual anatomy of a right SVC and left innominate vein, left subclavian vein access is preferred. In the region of clavicular depression, the skin, subcutaneous tissues, and clavicular periosteum are infiltrated with lidocaine. The needle, with attached syringe, is advanced gently through the skin until it contacts the clavicle, then it is “walked” under the clavicle. The needle must be oriented anteriorly to avoid entering the subclavian artery or the apex of the lung. Once under the clavicle, the needle is advanced slowly with constant gentle aspiration until a steady venous blood return is obtained. Using fluoroscopic guidance, the soft end of a wire is advanced through the vein into the right atrium (or the pulmonary artery in a cavopulmonary anastomosis). Due to negative intrathoracic pressure, air will be sucked into the vein if the needle is left open to air or if there is a mismatch between the size of the needle lumen and the wire diameter, especially if the patient is breathing spontaneously. Because of the risk of intravascular air, a sheath with a hemostasis valve is always used.
Umbilical Approach
Umbilical venous access is generally possible until the third day of life. The umbilical artery may be cannulated up to 1 week of age. It is best to avoid maneuvering a wire directly through the umbilical vessels. Often, infants arrive in the cath lab with umbilical lines in place. An indwelling umbilical vein catheter with its tip in the right atrium can be exchanged over a 0.018- to 0.021-in guidewire for a sheath and dilator; the sheath should be long enough to cross the ductus venosus and just enter the right atrium, but not so long as to puncture the right atrium. An indwelling umbilical arterial line may be exchanged over a wire; however, the circuitous course of this vessel makes catheter exchanges difficult. The use of a sheath in the umbilical artery is optional.
Catheter manipulation is difficult from the umbilical vessels. An umbilical venous catheter is directed toward the roof of the left atrium, across the PFO. Directing it to any other location will usually require the use of a deflecting wire. With an umbilical arterial catheter, the initial inferior loop that the umbilical artery takes before joining the internal iliac artery limits the ability to manipulate the catheter. It is important to use very gentle technique during cardiac catheterization in the newborn heart, as the cardiac walls are very thin—especially in the atria and left ventricular apex—and the chambers are small. Since small sheaths and catheters (3 and 4 Fr) can be placed in the femoral vessels, the benefits of improved catheter manipulation make a femoral approach preferable in most cases, even when the umbilical vessels are available.
Hepatic Approach
In the mid to late 1990s, transhepatic venous access was first described as an alternative to traditional venous access sites in limited clinical situations (10,11). When femoral, jugular, or subclavian venous access to the right atrium is not possible, a hemostatic sheath can be placed within a hepatic vein. This is particularly useful for device closure of an atrial septal defect when the IVC is interrupted or thrombosed. Overall, however, transhepatic venous access is infrequently used.
After the appropriate sterile preparation and local anesthesia (including infiltration of into the hepatic parenchyma), the skin is punctured along the mid to anterior axillary line at the subcostal margin using a long 21- or 22-gauge needle with or without an obturator. The needle is directed posteriorly, superiorly, and medially, toward the left shoulder. Historically, the needle is advanced under fluoroscopic guidance, but some have advocated for ultrasound-guided access (12). When the tip of the needle is approximately 1 cm from midline, the obturator is removed. Blood return with or without gentle aspiration suggests that the needle is in the vascular space. Positioning is then confirmed with a contrast injection; if contrast flows to the heart, the needle is in an appropriate hepatic vein, whereas if contrast flows into the liver parenchyma, the needle is in a portal vein and should be repositioned. Once the needle is found to be within an adequately sized hepatic vein, a 0.018-in wire is passed through the needle into the right atrium or SVC. With the needle removed, a stiff introducer is advanced over the wire, which is then exchanged for a larger 0.035- or 0.038-in wire. The introducer is then exchanged for the necessary hemostatic sheath with the tip of the sheath positioned in the low right atrium.
Catheter manipulation through hepatic venous access may be more troublesome than traditional femoral venous access. Directing catheters into the right ventricle and pulmonary arteries frequently requires creative use of preshaped catheters and deflecting wires.
Hepatic venous access also carries the risk of some unique complications, including intraperitoneal bleeding, hemobilia, gall bladder perforation, portal vein thrombosis, and liver abscess or peritonitis. Closure of the hepatic tract following sheath removal may depend on the need for ongoing anticoagulation, size of sheath used, and hemodynamic status; often hemostasis may be obtained with pressure (13).
Catheters and Wires
Functioning competently in the congenital cardiac catheterization lab requires an understanding of the various equipment and tools available to the cardiologist. Specifically, the cardiologist needs a practical familiarity with the different catheters and wires available in the cath lab.
For the most part, catheters are hollow, allowing transmission of pressure measurements, sampling of blood, and infusion of medications or contrast. In general, catheters can be classified as end-hole or side-hole. For the most part, end-hole catheters are used for hemodynamic measurements, blood gas sampling, and contrast injection into smaller vessels by hand. Angiographic injections are primarily performed using side-hole catheters. Catheters may be straight or shaped. Figure 16.1 illustrates some examples of different catheter shapes.
Right heart catheterization is typically performed using soft, balloon-tipped catheters. An end-hole balloon wedge catheter is used for hemodynamic pressure measurement and blood gas sampling. When the balloon is inflated with a small volume of CO2 and with small amount of manual torque, the catheter will follow the flow of venous blood into right atrium, across the tricuspid valve into the right ventricle and from the right ventricle into the pulmonary arteries. With the balloon-tipped end-hole catheter positioned in a distal branch pulmonary artery, gentle inflation of the balloon allows measurement of pulmonary artery wedge pressure. Angiography of the right heart is usually performed using a balloon-tipped angiographic catheter, which has side holes proximal to the balloon.
Left heart catheterization is typically performed using smaller caliber, thin-walled, but more rigid, catheter. A pigtail catheter is advanced to the descending aorta over a wire. With the wire removed, the catheter end is curled, allowing it to be advanced and withdrawn in the aorta without engaging smaller branch arteries. However, in order to advance the pigtail catheter into the left ventricle, a soft, typically tight-J–tipped wire is used to cross the aortic valve to prevent leaflet damage. Pressure measurements, blood sampling, and angiography can all be performed using the pigtail catheter.
Wires can be used to direct or stabilize catheters. Like catheters, wires come in many different diameters. Most wires have a soft distal end, which comes in various contours, including straight, J-tipped, and angled. Wires advanced through hollow catheters are used to probe and enter vessels that may be otherwise difficult to access with the catheter alone, such as stenotic branch pulmonary arteries or tortuous collateral vessels. Wires can also be used to cross a PFO for access to the left atrium and pulmonary veins. Stiff wires are used to stabilize catheters for angioplasty and valvuloplasty. Stiff, extra-long wires are valuable for maintaining position while exchanging one catheter for another.
Many other catheters and wires of various sizes, lengths, and contours are available for use depending upon the specific clinical scenario, but an expansive discussion is beyond the scope of this chapter.
Catheter Manipulation
A detailed discussion of catheter manipulation is beyond the scope of this chapter, but several points deserve mention. In the neonate and infant, cardiac tissue is thin. Perforation can occur easily, especially in the atrial appendages, right ventricular outflow tract, left ventricular apex, and aortic valve cusps. The risk of perforation can be decreased by gentle catheter manipulation, using small movements, the use of balloon catheters and floppy-tipped wires, and a thorough understanding of the cardiac anatomy and the desired catheter route and destination. The importance of reviewing all previous imaging studies before the catheterization cannot be overemphasized. If a catheter is too straight to manipulate to the desired location (e.g., across the tricuspid valve), it may be safely curved outside the body, in a hepatic vein, or using a tip-deflecting or shaped wire, rather than within the heart. The small catheters used
in infants and children can be damaged by vigorous manipulation. Thin-walled catheters, such as pigtails, should be advanced over a wire. Large catheter loops in the atrium or right ventricular outflow tract can cause hemodynamic instability owing to reflex bradycardia or tricuspid valve insufficiency; therefore, one needs to pay attention to all parts of the catheter, not just the tip. It is possible to tie an overhand knot with a catheter that has a large loop.
in infants and children can be damaged by vigorous manipulation. Thin-walled catheters, such as pigtails, should be advanced over a wire. Large catheter loops in the atrium or right ventricular outflow tract can cause hemodynamic instability owing to reflex bradycardia or tricuspid valve insufficiency; therefore, one needs to pay attention to all parts of the catheter, not just the tip. It is possible to tie an overhand knot with a catheter that has a large loop.
Collection of Hemodynamic Data
The purpose of a diagnostic heart catheterization is to collect data that will be used to define the patient’s condition and help make management decisions. The calculations made using data obtained during catheterization assume that blood samples and pressure measurements were obtained at a steady physiologic state. Changes in oxygenation, ventilation, heart rate, and blood pressure will directly affect these calculations and may produce inaccurate data that, in turn, can result in suboptimal patient management. Because a true “steady state” is rarely encountered in the cath lab, it is important to recognize any changes in heart rate, blood pressure, and oxygen saturation and how these changes will affect the accuracy of the data. The patient’s condition, oxygen saturation (or blood gas) samples, and hemodynamic data must be continually assessed for validity. The operator should be constantly aware of the patient’s hemodynamic state, and inconsistencies in the data must be clarified before the catheters are removed. Initial interpretation of all data obtained should be performed before leaving the cath lab to be certain that the information deemed necessary has been obtained. Although separated for purposes of discussion, the collection of hemodynamic/pressure data and oxygen sampling occurs simultaneously during the initial phases of the catheterization procedure.
Diagnostic heart catheterization typically begins with assessment of the initial arterial blood pressure, venous filling pressures, and measurements of oxygen saturations in the SVC and femoral artery. These data provide immediate information about the patient’s initial hemodynamic status, which is a crucial aspect of the procedure. One needs to assess initial blood pressure to be sure that the hemodynamic data recorded will be valid. Detection of shunts and quantifying resistance depends on the measurement of various flow indices, and for the purposes of this discussion, oxygen content (saturation) will be used as the primary indicator with respect to these calculations (see further discussion below). Children with an SVC saturation <60% or arterial saturation <70% have limited cardiac reserve. All factors influencing their conditions (i.e., body temperature, ventilatory status, acid–base balance, and volume status) should be assessed at the start of the procedure and maintained at an optimal steady state during the procedure. Since variation in any of these factors can affect the data obtained during catheterization, all procedures should be completed as expeditiously as possible.
Any abnormal data must be verified promptly in the cath lab. Abnormal pressure tracings should be compared to those expected or to baseline, and transducers recalibrated frequently to be sure they are properly zeroed. When sampling saturations, for instance, elevated pulmonary artery saturation should prompt the operator to identify whether the catheter was partially wedged or whether there is a left-to-right shunt. In addition, a significant “step-up” in saturation between consecutive cardiac chambers suggests the presence of a left-to-right shunt.
After collecting all saturations and pressures, and before proceeding to angiography, it is important to assess the pulmonary and systemic flows, resistances, and flow ratios. Systemic flow index can be estimated by recognizing that an arterial–venous (A–V) oxygen (O2) saturation difference of 20% to 25% corresponds to a “normal” cardiac index of approximately 3.5 L/min/m2. A larger A–V O2 difference correlates with a lower cardiac index. In addition, for a given A–V O2 difference, the higher the hemoglobin level, the lower the flow index (Fig. 16.2). A simple formula to estimate pulmonary flow index (QP) compared to systemic flow index (QS) is the ratio of pulmonary A–V O2 divided by systemic A–V O2. Details of calculations for flow indices and resistance are discussed later in this chapter.
Measurement of Hemodynamic Variables
The primary goals of any detailed hemodynamic catheterization are to measure pressures in all of the pertinent cardiac structures/chambers and to obtain oxygen samples in these structures in order to calculate systemic and pulmonary flow indices and vascular resistance.
Pressure Measurements
In the cath lab, pressures are most often measured using fluid-filled catheters connected to pressure-sensitive transducers. The change in pressure (force/unit area) in the cardiac chamber or vessel is transmitted along a column of incompressible fluid (saline or blood) contained within a nonexpansile tube (catheter) to a transducer. The transducer contains a diaphragm that moves a small distance (in a linear fashion) in response to change in pressure. The movement of the diaphragm is transmitted to an electronic strain gauge. The strain gauge converts pressure changes into voltage changes. These voltage changes are then converted into an electric signal, which is displayed as a waveform over time on a computer monitor. Systolic and diastolic pressures are measured instantaneously as the peak and trough, respectively, of the waveform. A mean pressure is obtained by electronic damping of the signal over several cardiac cycles. The system is calibrated to a zero point at level of the center of the heart. It is important to understand potential sources of error and artifact in the pressure tracings obtained. Two important concepts are frequency response (the ratio of output amplitude to input amplitude over the range of frequencies of the input pressure wave) and damping (the dissipation of the energy of oscillation of a pressure measurement system). Inaccurate pressure waveforms frequently are related to deterioration of the frequency response or to overdamping or underdamping. Other references contain a more complete discussion of these principles (14). Eight potential sources of pressure tracing artifact and recommendations for identifying the source of artifact and error are listed below:
Loose connections in the system: These usually result in an overdamped tracing. Backup of blood into the transducer tubing is an indication of a loose connection.
Air in the system: This is probably the most common cause of measurement error. Air may be introduced into the system at any of the connections, or dissolved air may come out of the saline used to flush the system. Air is compressible and its presence in the system lowers the frequency response. As a result, information inherent in the applied pressure wave is lost, producing what is commonly referred to as a damped tracing. Another indication of air in the system is the amplification of high-frequency input, producing overshoot or “fling” in the tracing. The appearance of a small amount of blood with obvious pulsatility in the transducer tubing is an indication that there is air in the system.
Inaccurate calibration or baseline drift: Even if the transducers are properly calibrated or “zeroed” at the beginning of the procedure, movement of either the patient or the transducers, or electric drift of the baseline, may result in inaccurate pressure recordings. Although small errors in calibration may be inconsequential in arterial recordings, they can have a significant impact in the measurement of venous pressures and pulmonary vascular resistance.
Partial catheter obstruction: This is usually the result of the catheter clotting or kinking. This usually occurs when small or thin-walled catheters are used. If blood is allowed to remain in the catheter lumen for any length of time, deposition of fibrin or platelets will reduce the lumen size, decreasing the frequency response.
Catheter “fling”: The appearance of fling (a tall, narrow spike) on a pressure recording has many causes. Overshoot, produced by air in the system, has been discussed. Rapid movement of the catheter tip, which may occur if the tip lies in a turbulent jet, can result in superimposition of high-frequency oscillations on the pressure recording. If the catheter is contacted by a cardiac structure (such as the mitral valve), the superimposed oscillation can alter the waveform dramatically. At times, these conditions are unavoidable. To minimize this error, use the mean systolic pressure rather than the peak systolic pressure, or inject a small amount of blood or contrast media in the tubing to intentionally damp the system.
End-hole artifact: When a column of blood stops suddenly against an end-hole catheter, kinetic energy is transformed into pressure energy, and the recorded pressure is falsely elevated. Similarly, when a column of blood is moving away from an end-hole catheter, the pressure recorded will be less than the true intravascular pressure, in proportion to the velocity of flow. This explains why the pressure in a stenotic proximal pulmonary artery has a lower peak systolic pressure than the distal larger vessel.
Peripheral pulse wave amplification: Both the peak systolic pressure and the pulse pressure are amplified with increasing distance from the aortic valve. The mean pressure, however, remains the same. This phenomenon is demonstrated by recording the pressure tracing during a pullback around the aortic arch, where the systolic pressure increases from the ascending to the descending aorta. While peripheral pulse wave amplification is a physiologic phenomenon and not an artifact, per se, it may lead one to incorrectly interpret pressure data. When comparing the ascending aorta systolic pressure with the femoral or radial artery systolic pressure, failure to take into account pulse wave amplification will result in underestimation of a pressure gradient across an aortic valve or coarctation of the aorta.
Catheter entrapment: An end-hole catheter placed in a small or heavily trabeculated ventricle can trap a small volume of fluid, resulting in an exaggerated pressure elevation during systole. This error can be eliminated by slightly withdrawing the catheter or deflating the balloon (if a balloon-tipped catheter is used). If these maneuvers are not possible, the pressure should be recorded using a side-hole catheter, provided that all of the side holes are within the chamber or vessel of interest.
Transducer-tipped wire catheters allow direct pressure measurement without relying on the propagation of fluid waves through a fluid-filled catheter (15). Such wire catheters consist of a floppy wire end distal to the transducer, which prevents the manometer from being damaged or puncturing the vessel or chamber wall. The pressure wire is connected to an analyzer system that displays numeric pressures and waveforms on a monitor. The pressure wire is introduced through an end-hole catheter. When the manometer reaches the end of the catheter, the wire and catheter pressures are calibrated on the monitoring system. The pressure wire is then advanced from the catheter into the vessel or chamber in question. Pressure wire manometry allows simultaneous pressure measurements in two contiguous locations, such as simultaneous ascending and descending aorta pressures in a patient with coarctation of the aorta. This technique may also be used to carefully record the pressure differential across a mechanical valve, with very gentle manipulation of the wire across the prosthetic valve opening. In the case of an aortic prosthesis, the retrograde catheter remains in the ascending aorta, whereas the pressure wire is advanced retrograde across the valve into the left ventricle. The thin pressure wire is also useful for obtaining pressure measurements distal to narrow communications that may be difficult to access with a catheter alone, such as the pulmonary artery pressure in child whose sole source of pulmonary blood flow is via a modified Blalock–Thomas–Taussig shunt (16).
Right Heart Catheterization
Characteristic right heart waveforms are shown in Figure 16.3 and normal hemodynamic pressure values for children are shown in Table 16.1. There are three characteristic waves in the right atrial tracing; a, c, and v waves. The a wave represents atrial systole and occurs just after the P wave on the electrocardiogram. The c wave is due to either right ventricular contraction or tricuspid valve closure, whereas the v wave results from filling of the right atrium against a closed tricuspid valve. The decrease in pressure after the a wave is the x descent, which is due to atrial relaxation. The decrease in pressure after the v wave is the y descent, which represents the opening of the tricuspid valve in early diastole.
Normally, the right atrial a wave exceeds the v wave by 2 to 3 mm Hg, neither wave exceeds 8 mm Hg, and both waves are within 5 mm Hg of the mean right atrial pressure. An elevated a wave is seen in the context of restricted right ventricular filling, such as tricuspid stenosis or a noncompliant right ventricle (e.g., pulmonic stenosis, pulmonary atresia, or pulmonary hypertension). The so-called “cannon” a waves may be seen with certain arrhythmias, when atrial contraction occurs after right ventricular contraction has closed the tricuspid valve. Elevated v waves are seen with tricuspid regurgitation, Ebstein anomaly, or a left ventricular-to-right
atrial shunt. The right atrium and venae cavae are compliant structures that can accept a large volume of blood with minimal change in pressure; thus, the v wave may not necessarily be elevated in the aforementioned conditions. Elevation of the mean pressure is seen with any condition that elevates the a wave or v wave, or in the context of myocardial failure. Low right atrial pressure (<1 to 2 mm Hg) suggests hypovolemia. Marked variability of the a wave and mean pressure may be seen with atrioventricular dissociation or with obstructive airway disease. A patient who is breathing spontaneously but has significant airway obstruction may have negative intrathoracic pressure and subatmospheric right atrial pressure, which may result in the introduction of air into the system if the catheter is not properly connected or the hub is maintained below the level of the chamber.
atrial shunt. The right atrium and venae cavae are compliant structures that can accept a large volume of blood with minimal change in pressure; thus, the v wave may not necessarily be elevated in the aforementioned conditions. Elevation of the mean pressure is seen with any condition that elevates the a wave or v wave, or in the context of myocardial failure. Low right atrial pressure (<1 to 2 mm Hg) suggests hypovolemia. Marked variability of the a wave and mean pressure may be seen with atrioventricular dissociation or with obstructive airway disease. A patient who is breathing spontaneously but has significant airway obstruction may have negative intrathoracic pressure and subatmospheric right atrial pressure, which may result in the introduction of air into the system if the catheter is not properly connected or the hub is maintained below the level of the chamber.
TABLE 16.1 Normal Hemodynamic Pressure Measurements in Children | ||||||||||||||||||||||||||||
---|---|---|---|---|---|---|---|---|---|---|---|---|---|---|---|---|---|---|---|---|---|---|---|---|---|---|---|---|
|
The right ventricular pressure tracing has a rapid upstroke during isovolumic contraction, a plateau during systolic ejection, a decline to near zero during isovolumic relaxation, and a slow rise to the end-diastolic pressure during diastolic filling. Normally, the peak systolic pressure is <30 mm Hg, and the end-diastolic pressure is <8 mm Hg. Except in the context of tricuspid valve stenosis, the end-diastolic pressure corresponds to the right atrial a wave. Right ventricular systolic pressure is elevated in the presence of a large ventricular septal defect, right ventricular outflow tract obstruction, and pulmonary hypertension. Recordings should be obtained in the apex and in the outflow tract to confirm the absence of any intracavitary gradient.
The normal pulmonary artery systolic pressure is equal to the right ventricular systolic pressure (<30 mm Hg), and the mean pressure is <20 mm Hg. Pulmonary artery diastolic pressure begins with the dicrotic notch caused by valve closure, and the diastolic pressure is typically no more than 2 to 3 mm Hg higher than the wedge pressure. Increased pulmonary artery pressure occurs with distal obstruction to flow, such as with peripheral pulmonary stenosis, pulmonary arteriolar obstruction, pulmonary thromboembolism, pulmonary venous obstruction, or left atrial hypertension (due to other causes such as cor triatriatum, mitral stenosis, or left ventricular diastolic dysfunction). Pulmonary artery pressure is also elevated in the presence of aorta-pulmonary artery communications (such as patent ductus arteriosus or aortopulmonary window) or a significant ventricular septal defect (if there is no pulmonic stenosis). In the presence of pulmonary hypertension, a pulse pressure of <40% of the peak systolic pressure suggests a fixed resistance, whereas a wide pulse pressure (>60% of the peak systolic pressure) suggests high flow and low resistance. Pulmonary artery diastolic pressure that is significantly higher than mean left atrial or pulmonary artery wedge pressure also suggests an obstructive cause of pulmonary hypertension.
Systolic pressure gradients between right ventricle and pulmonary artery are due to right ventricular outflow tract obstruction, although gradients of 5 mm Hg may be normal. Gradients ≤10 mm Hg may be seen with structurally normal pulmonary valves and increased blood flow, as with a large atrial septal defect. In the setting of very severe branch stenosis or a very tight pulmonary artery band, the catheter may produce enough obstruction of the vessel as it crosses the stenosis that the pressure distally resembles the wedge pressure tracing.
Pulmonary artery wedge pressure is usually a good reflection of the left atrial and left ventricular end-diastolic pressures because of the absence of valves in the pulmonary circulation. When an end-hole catheter is appropriately wedged in a pulmonary artery branch, the distal pulmonary venous pressure is transmitted retrograde through the capillary bed and arterioles to the catheter tip. When using a balloon-tipped wedge catheter (end-hole), the catheter is advanced as far as it can into the distal pulmonary artery and the balloon is partially inflated while monitoring the tracing for its characteristic appearance. The wedge tracing should have the characteristic a- and v-wave appearance of an atrial tracing (see Fig. 16.3). The wedged catheter position is confirmed by observation of the characteristic left atrial waveform or by withdrawal of fully saturated blood. When small catheters are used, it may not be possible to withdraw a blood sample from the wedged position. Interpretation of the wedge pressure must be guided by an understanding of the anatomy. The pulmonary artery wedge pressure does not reflect the left ventricular end-diastolic pressure when there is pulmonary vein stenosis, cor triatriatum, mitral stenosis, or anomalous pulmonary venous return. When the wedge pressure is elevated, these lesions should be confirmed or ruled out by direct measurement of the left atrial or left ventricular end-diastolic pressure.
Left Heart Catheterization
Characteristic left heart waveforms are shown in Figure 16.4 and normal pressure values are shown in Table 16.1. The normal left atrial mean pressure is 6 to 10 mm Hg (depending on age), which is several mm Hg higher than the right atrial mean pressure. In contrast to the right atrium, the left atrial v wave is usually higher than the a wave, and neither is >5 mm Hg above the mean pressure. An elevated a wave is seen with defects resulting in left atrial outflow obstruction (mitral stenosis, supravalvar mitral ring) or with diseases that impair left ventricular compliance (aortic stenosis, coarctation of the aorta). The a wave may be dominant with an atrial septal defect, as a large atrial septal defect allows transmission of pressure across the septum, or with diseases that elevate the right atrial a wave. Elevated v waves typically occur with mitral regurgitation. Elevation of the left atrial mean pressure (and both the a and v waves) may be encountered with large left-to-right shunts at the ventricular or great vessel level or as a sign of left ventricular failure. If the end-diastolic pressures in the left atrium and left ventricle are not equal, some form of mitral valve obstruction is present. Higher gradients (>8 to 10 mm Hg) suggest structural mitral stenosis, whereas lower gradients suggest physiologic stenosis due to increased blood flow across the valve, such as from a large ventricular septal defect. When transseptal technique is used to enter the left atrium, one can use a smaller diameter catheter to advance into the left ventricle and simultaneously measure left atrial and left ventricular pressure.
The peak systolic pressure in the left ventricle should be equal to or up to 5 mm Hg greater than the peak systolic pressure in the ascending aorta. A gradient between the left ventricle and the aorta is present in dynamic left ventricular obstruction (as in hypertrophic cardiomyopathy), subaortic stenosis, or aortic valve stenosis. The normal aortic pressure is a reflection of left ventricular stroke volume and systemic vascular resistance. Near the aortic valve, the arterial waveform displays a relatively slow upstroke, a broad peak, and a near-linear drop to end-diastole. In the distal arteries, the peak becomes sharper, the dicrotic notch (representing the decrease in pressure with closure of the aortic valve) becomes more obvious, and pulse wave amplification occurs.
The pulse pressure in the ascending aorta is usually 25 to 50 mm Hg, or <50% of the peak systolic aortic pressure. Increased pulse pressure and low diastolic pressure may be seen with aortic insufficiency, a ruptured sinus of Valsalva, or conditions in which there is a low-resistance diastolic runoff such as patent ductus arteriosus, aortopulmonary collaterals, aortopulmonary window, truncus arteriosus, and systemic AV fistula. A narrow pulse pressure may be encountered in pericardial tamponade or low cardiac output states. A gradient between the ascending and descending aorta suggests coarctation of the aorta.
Derived Hemodynamic Variables
Measurement of cardiac output, in terms of pulmonary and systemic blood flow, is a necessary first step to quantifying shunt volume and vascular resistance. Because cardiac output cannot be measured directly, it can be estimated using the indicator dilution technique described by Fick (17). The indicators most commonly used are oxygen or cold saline (thermodilution) (18). Various analogies have been used to explain the concept of the Fick principle. In one analogy, a coal-bearing train, representing blood, passes through a coal-loading station (capillary bed) at a constant but unknown rate (cardiac output). The train consists of a series of cars (hemoglobin), each of which have a known load of coal (oxygen content). By knowing the rate of delivery of the coal (oxygen uptake) and the amount of coal in the cars before and after the station, one can easily calculate the rate at which the train is moving through the station. In quantitative terms, cardiac output can be calculated according to the following logic:
Oxygen in the Blood
Oxygen is the most common indicator that is used for purposes of calculating flow and resistance in the congenital cardiac patient. Because the quantity of oxygen in the blood that is being sampled directly affects the calculation of cardiac flow rates, it is important to be able to accurately measure or estimate the oxygen content of a blood sample.
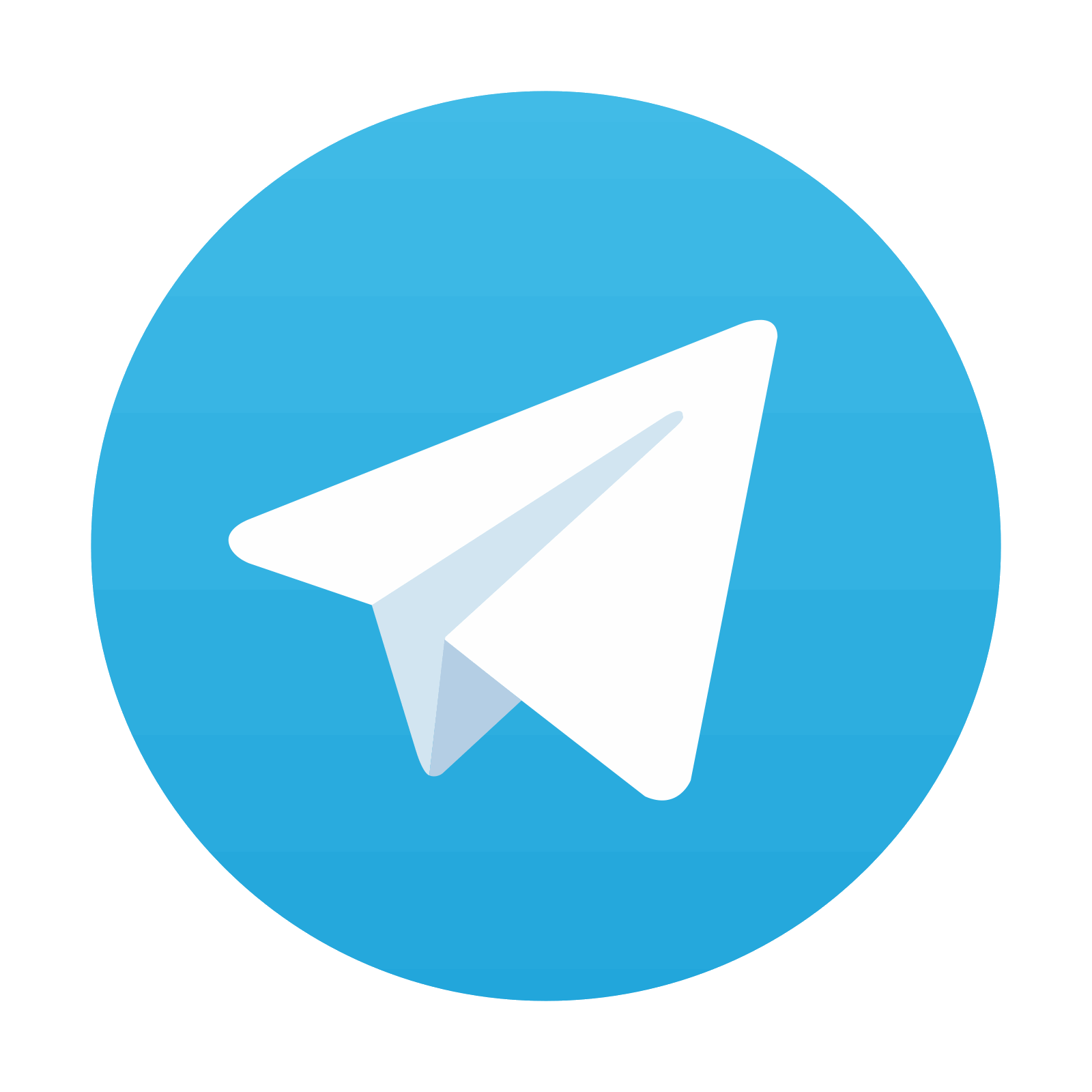
Stay updated, free articles. Join our Telegram channel
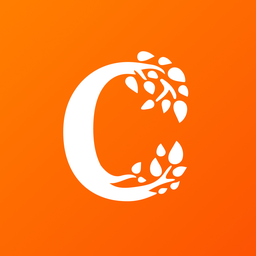
Full access? Get Clinical Tree
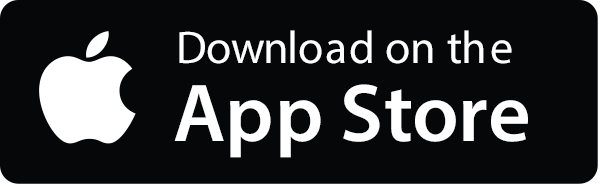
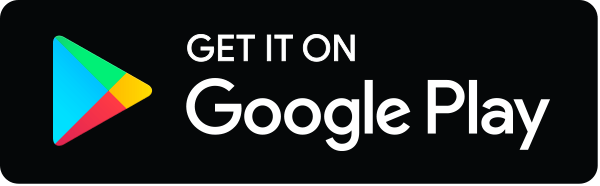
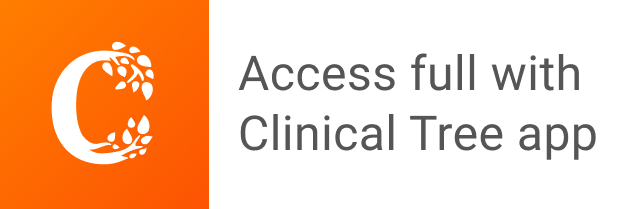