Background
Several treatment options are available for pulmonary vascular disease, and more patients are considered for right heart catheterization. The aims of this study were to evaluate the diagnostic ability of echocardiography to detect pulmonary hypertension and increased pulmonary vascular resistance (PVR).
Methods
This retrospective study comprised 118 patients investigated within 48 hours of right heart catheterization. Echocardiography was used to assess pulmonary artery systolic pressure and pulmonary artery mean pressure, filling pressures, cardiac output, and PVR. To diagnose increased PVR, three echocardiographic variables related to pressure reflection in the pulmonary circulation were used. Separate cutoff values aimed at ruling in (high positive likelihood ratio [PLR]) and ruling out (low negative likelihood ratio) pulmonary hypertension (pulmonary artery mean pressure >25 mm Hg) and increased PVR (>3 Wood units) were determined from a derivation group ( n = 59, receiver operating characteristic curve analysis) and evaluated in a test group ( n = 59).
Results
The linear relations between hemodynamic variables assessed with simultaneous echocardiography and right heart catheterization were moderate to strong ( R = 0.55 to 0.95), and there were no significant differences, but the limits of agreement were wide. With Doppler pulmonary artery systolic pressure >39 mm Hg, the PLR for pulmonary artery mean pressure >25 mm Hg was 4.7, and with Doppler pulmonary artery systolic pressure ≤29 mm Hg, the negative likelihood ratio was 0.12. The PLR for pressure reflection variables with ruling-in cutoff values ranged from 4.3 to 6.4. With all three variables positive, the PLR was 9.9. The negative likelihood ratio with ruling-out cutoff values ranged from 0.22 to 0.08.
Conclusions
Echocardiography that includes assessment of pressure reflection in the pulmonary circulation can rule in and rule out pulmonary hypertension and increased PVR.
The development of right ventricular failure is an ominous sign that predicts adverse outcomes and influences treatment strategies in patients with cardiopulmonary diseases. Although right ventricular failure may be due to primary myocardial dysfunction, it is more often caused by pulmonary hypertension and increased right ventricular afterload. Compared with the left ventricle, the thin-walled right ventricle demonstrates increased sensitivity to afterload elevation. Pulmonary hypertension predict all-cause mortality in patients with both preserved and impaired left ventricular systolic function.
Low pulmonary vascular resistance (PVR), compliant arteries, and no reflection of the pressure wave characterize the normal pulmonary circulation. In clinical practice, the level of right ventricular afterload is most often described using the pulmonary artery (PA) pressure. The PA pressure is dependent on flow and resistance. The assessment of PVR, which reflects the transpulmonary pressure gradient divided by the pulmonary blood flow, is considered to require right heart catheterization (RHC).
Several specific treatment options are available for patients with pulmonary vascular disease. The diagnosis is difficult, and diagnostic delay is a well-known problem. In patients with pulmonary hypertension and normal left ventricular ejection fractions, is it not possible using standard echocardiography to distinguish increased pressure due to pulmonary venous hypertension from increased PVR. The treatment of pulmonary hypertension due to increased PVR in patients with left heart disease is controversial. However, the diagnosis of vascular disease is increasingly important in the era of heart failure treatment with mechanical support because it affects treatment strategies. As a consequence, more patients are considered candidates for RHC.
Much attention has been given to the development of echocardiographic methods to assess PA pressures and resistance to flow. PA pressures can be estimated using different noninvasive methods with good linear relations to invasive measurements. To estimate resistance to flow is more challenging because it combines several variables (PA pressure, pulmonary capillary wedge pressure [PCWP], and cardiac output). Surrogate variables for PVR have been proposed, but their usefulness is debated, and in recent American Society of Echocardiography (ASE) guidelines for assessment of the right ventricle, it is not recommended to estimate PVR for routine use. We found in previous studies that PVR could be calculated from echocardiographic data in patients with pulmonary arterial hypertension and that the presence of pressure reflection in the pulmonary circulation can be used to identify patients with increased PVR. In both these studies, the patient cohorts were biased toward pronounced pulmonary vascular disease with severely increased PVR. In the present study, we investigated the diagnostic ability of echocardiography to detect pulmonary hypertension and increased PVR in a population representative of those undergoing RHC at our institution.
Methods
Study Population
This retrospective study comprised 118 patients who were referred for clinical RHC between May 2009 and November 2012. The inclusion criteria were (1) regular cardiac rhythm, (2) RHC performed within 48 hours of echocardiography, (3) pulsed Doppler recording in the PA, and (4) tricuspid regurgitation that enabled the assessment of right ventricular peak systolic pressure from Doppler echocardiography. The study was approved by the ethics committee at the University of Gothenburg (448-10).
Hemodynamic Measurements
A Swan-Ganz catheter (7 Fr; Baxter Healthcare, Edwards Critical Care Division, Deerfield, IL) was introduced through the right internal jugular vein under fluoroscopic guidance using the Seldinger technique. The following variables were measured or derived: mean right atrial pressure, PA systolic pressure (PASP), PA mean pressure (PAMP), PCWP, cardiac output, and PVR.
Cardiac output was determined using the thermodilution method as the mean of three to five consecutive measurements not varying by >10%.
Pressure Reflection
In the normal pulmonary circulation, the pressure and flow waves generated by the right ventricle are almost completely dampened and display, therefore, similar contours. In individuals without obstruction in the right ventricular outflow tract (RVOT), the shape of the pressure waveforms in the right ventricle and PA (including the timing of peak pressure) are similar. Changes in the pulmonary precapillary properties cause a reflection of pressure and flow waves, with marked changes in wave contours. The reflected pressure wave will add on to the forward traveling wave, and there will be an increase in pressure in the right ventricle and PA while the flow is decreasing ( Figure 1 ). The site and magnitude of pressure reflection influence the timing of peak velocity in the RVOT, the timing of peak velocity in relation to peak pressure in the right ventricle, and the magnitude of pressure increase in the right ventricle after peak velocity in the RVOT ( Figure 1 ). In the present study, we assessed three variables in relation to PVR: (1) acceleration time (AcT): the time interval from opening of the pulmonary valve to peak velocity; (2) time from peak velocity in the right ventricular outflow tract to peak pressure in the right ventricle (tPV-PP): the time interval from peak velocity in the RVOT to peak velocity in the tricuspid regurgitant jet (peak pressure in the right ventricle); and (3) augmented pressure: the pressure increase (augmentation) in the right ventricle after peak velocity in the RVOT.

Figure 1 explains in a schematic drawing the pressure reflection phenomenon, including the three related variables, and Figure 2 describes how these variables are obtained using echocardiography.

Doppler Echocardiography
The left ventricular ejection fraction was measured using Simpson’s method or estimated by eyeballing. Mitral and pulmonary vein flow was recorded using pulsed Doppler from the tip of the valve and the upper right pulmonary vein. Tissue pulsed Doppler recordings were performed in the basal part of the left ventricular septum. The volume of the left atrium was estimated using the area-length method in end-systole. Right ventricular systolic function was assessed using the fractional area change method by tracing the end-diastolic and end-systolic areas (fractional area change = end-diastolic area − end-systolic area/end-diastolic area × 100) and by the tricuspid annular plane systolic excursion method by M-mode echocardiography. All Doppler measurements were performed offline with a sweep speed of 100 to 200 mm/sec, and the investigator was blinded to the results of the catheter investigation.
The assessment of pressure reflection requires continuous-wave Doppler recording of the tricuspid regurgitant jet and pulsed-wave Doppler recording in the RVOT. We used multiple windows guided by color Doppler to obtain the highest velocity. Most often, the highest velocity was found in a projection that showed the right ventricle somewhere between a standard four-chamber view and a parasternal view. The flow velocity was recorded by placing a 5-mm pulsed-wave Doppler sample volume in the RVOT. The timing of the pulmonary valve opening and the peak velocity was determined as the time from a reference point on the electrocardiographic QRS complex (most often the peak of the R wave) and the onset (a-b) and peak velocity (a-d) in the RVOT registered with pulsed-wave Doppler ( Figure 2 ). The timing of right ventricular peak systolic pressure was determined as the time from QRS to the peak velocity of the regurgitant jet (a-c) using the same QRS reference as for RVOT registration. In subjects in whom the peak velocity in the RVOT preceded the peak velocity (a-d < a-c) in the regurgitant jet, the time interval (a-d) was superimposed onto the velocity spectrum of the tricuspid regurgitant jet to calculate the pressure gradient corresponding to the peak velocity in the RVOT. The velocity across the tricuspid valve at this time interval was measured, and the pressure gradients between the right ventricle and the right atrium were calculated (pressure gradient = 4 × velocity 2 ). The mean right atrial pressure was estimated using the inferior vena cava dimension and collapsibility with inspiration. The tPV-PP was calculated as [(a-c)−(a-d)]. The time from the onset of flow in the RVOT to peak velocity (AcT) was calculated as [(a-d)−(a-b)]. The augmented pressure was calculated as the difference between peak pressure in the right ventricle and the pressure corresponding to peak velocity in the RVOT. In previously performed variability studies, the interobserver group variability (described by the coefficients of variation, expressed as the mean value of differences divided by the mean value of the two measurements) for measurements made on the same recording were 6%, 8%, and 9% for AcT, tPV-PP, and augmented pressure, respectively. The corresponding interobserver individual variability (the standard deviation of differences divided by the mean value of two measurements) was 7%, 9%, and 13%.
Grading of Left Ventricular Filling Pressure
The grading of left ventricular filling pressure was performed according to the recommendations of the ASE. In patients with depressed ejection fractions (<50%) and E/A ratios ≥2, the estimated Doppler PCWP used in the comparison with RHC and calculation of PVR was 20 mm Hg. If the PCWP was considered normal, we used 9 mm Hg in the comparison, and if the E/A ratio was pseudonormal and other supportive parameters (pulmonary vein S/D ratio, PASP, tissue Doppler) suggested increased PCWP, we used 15 mm Hg. In patients with normal ejection fractions, we used 20 and 9 mm Hg for those with increased and normal filling pressures on the basis of tissue Doppler findings. In patients with inconclusive tissue Doppler findings in whom other supportive parameters (volume of the left atrium, PASP) suggested increased PCWP, we used 15 mm Hg.
Calculation of PVR on the Basis of Echocardiographic Data
Stroke volume was calculated as the product of the cross-sectional area of the left ventricular outflow tract and the velocity-time integral. Mean PA pressure was calculated as follows :
PAMP Doppler = ( 0.65 × PASP Doppler ) − 1.2 mmHg .
PVR Doppler = ( PAMP Doppler − PCWP Doppler ) / ( stroke volume × heart rate ) .
PVR Abbas = ( TRV / VTI RVOT ) × 10 + 0.16.
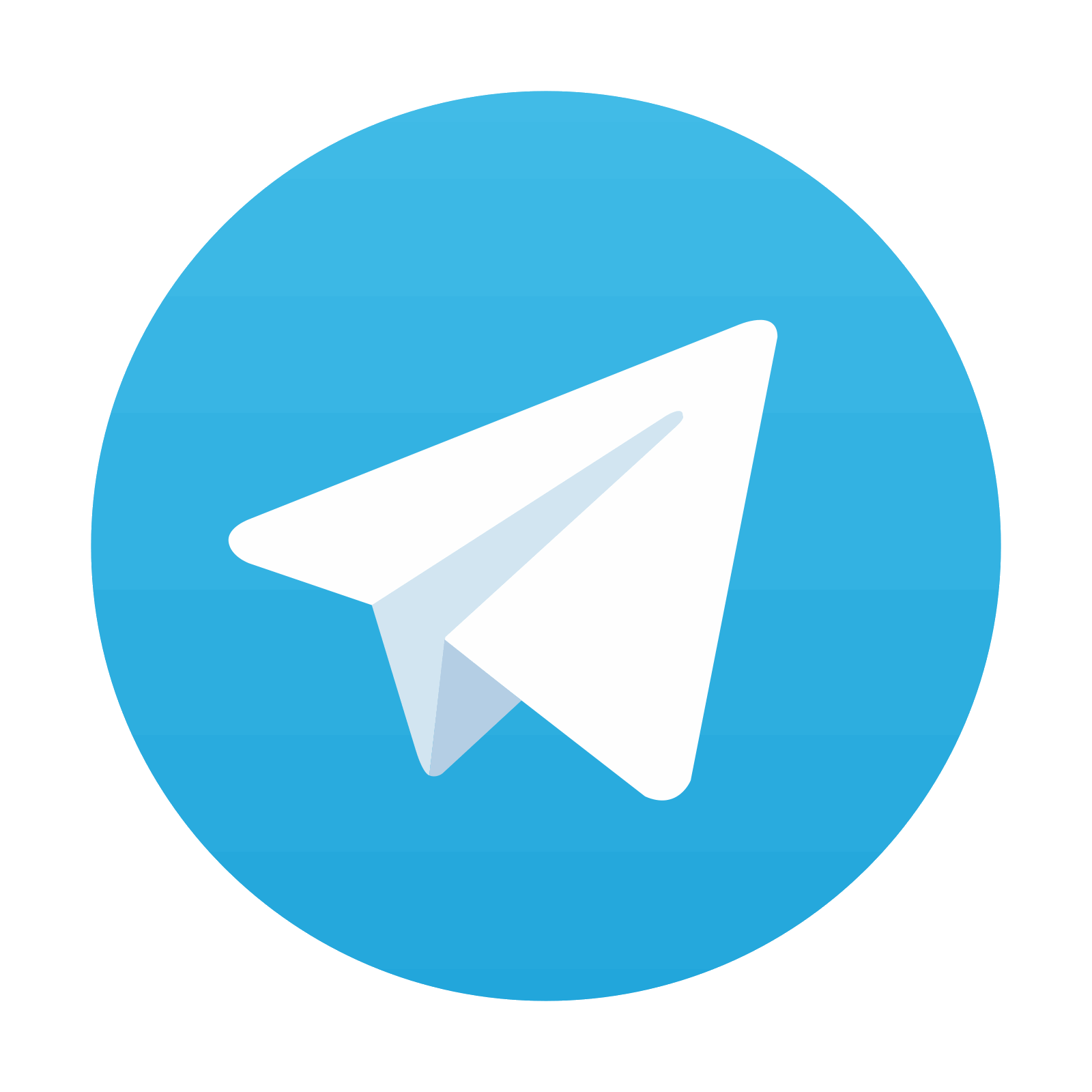
Stay updated, free articles. Join our Telegram channel
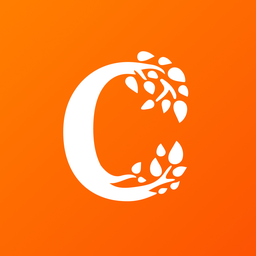
Full access? Get Clinical Tree
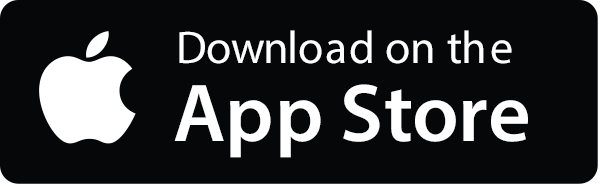
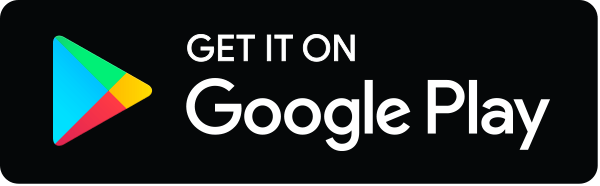