Keywords
Circulation in invertebratesCirculation in vertebratesAmphibian circulationCirculation in insectsCirculation in lanceletFish circulationBird circulationCross-current gas exchangeLymph circulationOpen circulationClosed circulationIn series circulationIn parallel circulationThe main function of the circulatory system is to uphold a specific inner environment, which according to the type, is essential for the life and development of the organism. This includes, but is not limited to, distribution of nutrients and removal of metabolic waste, immune defense, thermoregulation, reproduction, and transport of respiratory gases. There are two basic types of circulation. The open circulatory system of invertebrates is characterized by a system of body cavities or coeloms connected with vascular conduits, both of which lack an endothelial cell layer. This simple arrangement allows the circulating fluid, the hemolymph, to bathe the inner organs directly, thus fulfilling the dual function of tissue fluid and blood. We will see that in this respect it functionally resembles the early embryonic circulation of vertebrates before the formation of continuous vascular endothelium. In contrast, the closed circulatory system of the vertebrates is phylogenetically younger and consists of a network of continuous vessels in which the blood does not come in direct contact with the surrounding tissues. However, a significant overlap exists at the level of local circulations and individual organs within the vertebrate and invertebrate circulations. For example, while most of the capillary beds in the mammalian circulation are of the continuous type with tight endothelial junctions, the vascular sinuses of the bone, liver, and spleen are lined with fenestrated capillaries, readily permeable to plasma and large molecules (see Sect. 21.1). Technically, they too belong to the open circulation. Similarly, several classes of invertebrates such as, i.e., the Cephalopoda and the Oligochaeta have, in addition to coelomic spaces, continuous vascular loops which resemble the closed circulations of vertebrates.
11.1 Invertebrates
The circulatory systems in invertebrates are as diverse as the large phylum to which they belong, with numerous species having no heart and only a rudimentary circulatory system, in contrast to others, with well-developed hearts and highly responsive peripheral circulation. The circulation in the common earthworm (Lumbricus terrestris/oligochaeta) is typical of a large group of lower invertebrates. The large coelomic cavity is divided into some 150 compartments by externally visible septa. The degree of septal filling with coelomic fluid regulates the form and movement of the animal and serves as “hydraulic skeleton.” Although the circulating fluid in earthworms is referred to as blood, this is a misnomer, since the respiratory pigment, a large heme-carrying molecule (erythrocruorin is about 36 times larger than hemoglobin), is not contained within the erythrocytes, but floats freely in plasma. Moreover, the vascular basement membrane is located on the luminal side of endothelial cells which contain contractile elements.
It has been suggested that the basement membrane plays a similar role in keeping the heme molecules within the vascular system, as does the erythrocyte membrane in containing the hemoglobin [1]. There are no specialized organs of respiration in the earthworm, the skin being the only organ available for gas exchange.

Schematic representation of earthworm’s circulatory system (Lumbricus terrestris). The blood from segmental vessel is collected in the contractile dorsal vessel and flows toward the head pole of the animal. Five pairs of segmental vessels with thickened, muscular walls (lateral hearts) direct the blood into the ventral vessel and back toward the tail (blue and red arrows). In addition to transporting nutrients, the function of the blood and of the coelomic fluid is to maintain the internal fluid pressure, i.e., the hydraulic skeleton, essential for burrowing and locomotion. The pressure in the active (contractile) loop of the circuit is lower than in the non-contractile ventral vessel, suggesting that the source of blood propulsion lies within the capillaries, rather than in the contractile vessels themselves
To assess the contribution of the lateral hearts for the maintenance of the circulation, Johnston removed the frontal somites of worms and observed the survivors during the regenerative phase for several weeks. The vessels at the anterior part of the animal were “greatly crowded and distended with blood,” while the posterior parts remained relatively unfilled. Serial sections showed the greatest distension of the dorsal vessel and of the vascular plexuses of the intestinal wall, while the ventral vessel appeared normal in size [2]. Unfortunately Johnston did not comment on the level of activity in the surviving animals, but the experiment nevertheless points to the importance of the lateral hearts in directing the flow and in maintaining the pressure in the ventral vessel. The congestion of the dorsal vessel and of the capillary beds further suggest that the capillary flow continues in the original direction, in spite of the major disruption of the vascular integrity and a partial loss of the “hydraulic skeleton.”
The importance of intravascular pressure in maintaining mobility among invertebrates can be appreciated in the class of Cephalopoda, a group of very active animals, whose level of activity can be compared to that reached by vertebrates. They too, have a “closed” circulatory system, with vascular and extracellular compartments, averaging 5.6% and 28% of total body weight, respectively, a proportion similar to that in the vertebrates. Their level of metabolic activity is higher than that of other molluscs [6].

Circulatory system of a cephalopod (Octopus dofleini). Deoxygenated blood returns by vena cava and abdominal veins into the paired branchial hearts and is ejected into the gills. The oxygenated blood collects via efferent branchial vessels in the valve-less systemic ventricle and is expelled into the paired aortae. Direction of blood flow as indicated by arrows. (Reproduced from ref. [8], used with permission of John Wiley and Sons)
Johansen and Martin investigated circulatory dynamics during rest and exercise in the large octopus (Octopus dofleini) [9]. Simultaneous intravascular pressures in the aorta, branchial vessels, and vena cava were measured in unrestrained animals. Baseline aortic pressures were in the range of 55 cm H2O for systolic and 40 cm H2O for diastolic. The caval pressures (pulsatile synchronously with respirations) were between 2 and 15 cm H2O. The heart rate varies, but was in the range of 6–8 beats/min. During slow, short movement (less than a minute), the systolic pressure increased to 73 cm H2O and the diastolic pressure to 60 cm H2O, with the pulse pressure of 13–14 cm H2O, without any change in heart rate. During sustained exercise (3 min), the heart rate increased from 6 (at baseline) to 8 beats/min, and peak systolic pressure rose to 75 by the end of the exercise period. In the immediate post-exercise period, the diastolic pressures remained markedly elevated for 10–15 min before dropping back to baseline levels. The authors were at loss to explain the sudden increase of pressures at the onset of exercise, considering that there was little or no change in heart rate. This, together with the lack of valves would make the central ventricle an inefficient propulsion pump, at best. The authors invoke contraction of the general body musculature as a factor in sustaining the increased diastolic pressure during activity, a view also supported by Chapman [10]. However, this does not explain the curious observation that the systemic ventricle can stop beating for a considerable period (up to 2 h), without a significant drop in aortic pressure. Despite the fact that during asystolic periods an increased activity was noted in branchial hearts, this could not account for the sustained aortic pressure, since the hearts are placed in the venous limb, before the extensive capillary network of the ctenidia (gills). The authors evoke “a massive peripheral vasoconstriction” as a possible cause of sustained aortic pressure during periods of asystole, but do not provide evidence for its existence [9].
11.2 Tracheate Insects

(a) Insect circulatory system—schematic. Hemolymph enters the contractile dorsal vessel (aorta) via paired segmental vessels terminating with the ostia. The ostia open during alary muscle relaxation (diastole) to allow the flow of hemolymph into the dorsal vessel. The dorsal vessel flow continues cephalad, bathes the organs of the head, enters into sinuses along the thorax, and returns into the abdomen, before it re-emerges in the dorsal vessel. Arrows indicate the flow of hemolymph. (b) Diagram of the insect dorsal vessel (Periplaneta Americana L.). The walls of the heart consist of a single layer of multinucleated striated muscle cells. In spite of rich innervation, cardiac contractions are myogenic. The entire dorsal vessel contracts simultaneously, possibly interrupting the autonomous flow of hemolymph. (c) Detail of abdominal heart segment in a cockroach (Periplaneta americana L.) Arrows indicate flow direction of hemolymph. (d) Hemolymph flow reversal in adult blow fly (C. vicina). Tidal movement of hemolymph into the thorax (a) alternating with movement into the abdomen (b). In some insect and larval species, rapidly alternating tidal movements of hemolymph occurs, coupled with respiration and abdominal movement. The role of the heart in impelling the flow of hemolymph has been questioned. (Figures (b) and (c) adapted and fig (d) reproduced from ref. [12], used with permission of Elsevier)
The insect’s hemolymph is a thin plasma-like fluid consisting mainly of water, proteins, amino acids, sugars, and electrolytes. The cellular components known as the hemocytes represent only 10% of hemolymph’s total volume, and their function is clotting, cellular defense, and phagocytosis. In comparison, the cellular component in vertebrate plasma is significantly higher, up to 50%. The hemocytes do not contain hemoglobin or other oxygen carrying pigments, since a separate tracheal system exists for the delivery of oxygen. The flow of hemolymph in the dorsal vessel is generally in the direction from the abdomen toward the head where, emerging from the aorta, it bathes the organs of the head and flows caudally via the three abdominal sinus cavities until it is collected again in the heart.
In keeping with the time-honored biological notion that a rhythmically contracting heart equals a pump, the insect heart is likewise assumed to function as such. However, much like in the case of the vertebrate embryo, the control of insect circulation has been subject of renewed interest in recent years and several phenomena, which point to an autonomous movement of hemolymph, have been observed but, unfortunately, not recognized.
Over the past several years, the difficult question of primary neurogenic control of insects’ hearts has been resolved. In some species the heart has no innervation, and all myogenic cells clearly possess the pacemaker activity. In addition, there are numerous species with richly innervated heart tubes. It has been argued that those too exhibit autonomous activity when anatomically or chemically denervated [13].
The real difficulty with the purported pressure propulsion arises, when we consider the mechanism of hemolymph propulsion through the insect. In theory, the peristaltic contraction of the alary muscles forces the hemolymph from chamber to chamber, while during relaxation phase (diastole), the ostia open and allow the flow of hemolymph from the segmental vessels to refill the heart. However, the role of the dorsal heart as an effective organ of propulsion has been questioned, since the pressures generated in the abdomen are 100–500 times greater than those generated by the heart [14]. In some insects, such as in the ordinary house fly, the heart beat varies between 0 and 300, occurring irrespective of the insect’s resting state or of flight. More remarkably, the fly can sustain the metabolic activity at 100-fold its basal level, far surpassing any mammal [15]. In comparison, the exercising dog can increase its metabolism up to 40 times and world class athletes by about 20 times [16]. This raises the question of the mechanism involved in the almost instantaneous overcoming of the fluid inertia, every time the flow of hemolymph comes to a halt. To make the matter even more complex, there is the peculiar phenomenon of hemolymph flow reversal (observed already by Malpighi in the seventeenth century), which occurs in several species of flies, and in the pupal stages of a number of insects [12, 17] (Fig. 11.3d). Given such complex “to and fro” movements of the hemolymph, how can the heart act as a bidirectional pump, and what would be its possible control mechanism, given the fact that the heart exhibits autonomous (myogenic) contractions in response to stretch and filling volume? Should the heart work as a peristaltic pump, as the theory proposes, how can one account for the pumping mechanism of the cockroach heart, for example, whose dorsal vessel contracts simultaneously along its entire length, rather than peristaltically as is the case in other insects? (Fig. 11.3b) [12]. The concept of pressure-driven circulation implies that the system of vessels is sufficiently tight to withstand the pressure generated by the circulating pump. Such a system is clearly not possible in the case of an open circulation with its system of coelomic spaces, which would soon become filled with excess amount of circulating fluid. Moreover, vessels devoid of the basement membrane and tight endothelial junctions would not be effective in containing the pressurized hemolymph. Given the fact that the circulation of the insect is closely coupled with its metabolic activity and, to some extent, with the environmental temperature, it becomes clear that the movement of the hemolymph is the expression of the metabolic activity of the insect itself and that the heart is inserted in the circuit in order to check or impede its flow. It is evident that, as in the case of vertebrates, the primary control of the insect circulation is metabolic and that the heart plays a secondary role, rhythmically restraining the flow of hemolymph.
11.3 Early Vertebrates

(a) Schematic diagram of a sessile adult tunicate, sea salp with an open circulatory system. Part of the mantle removed to show the inner structure. The tubular heart consists of single atrium and ventricle, has no structural valves, and is enveloped by the pericardial fold. Myogenic peristaltic contractions are initiated by pacemaker cells at each end of the heart and occur at the rate of about 20 times per minute. (Adapted from ref. [20], used with permission of Springer Nature). (b) Linear diagram of the path the blood traverses during the forward flow phase in sea salp (Thalia democratica). The blood flows along the vessels which lack endothelium to bathe various organ systems. It reverses after a pause and flows in the opposite direction. (Reproduced from ref. [19], used with permission of Cambridge University Press)
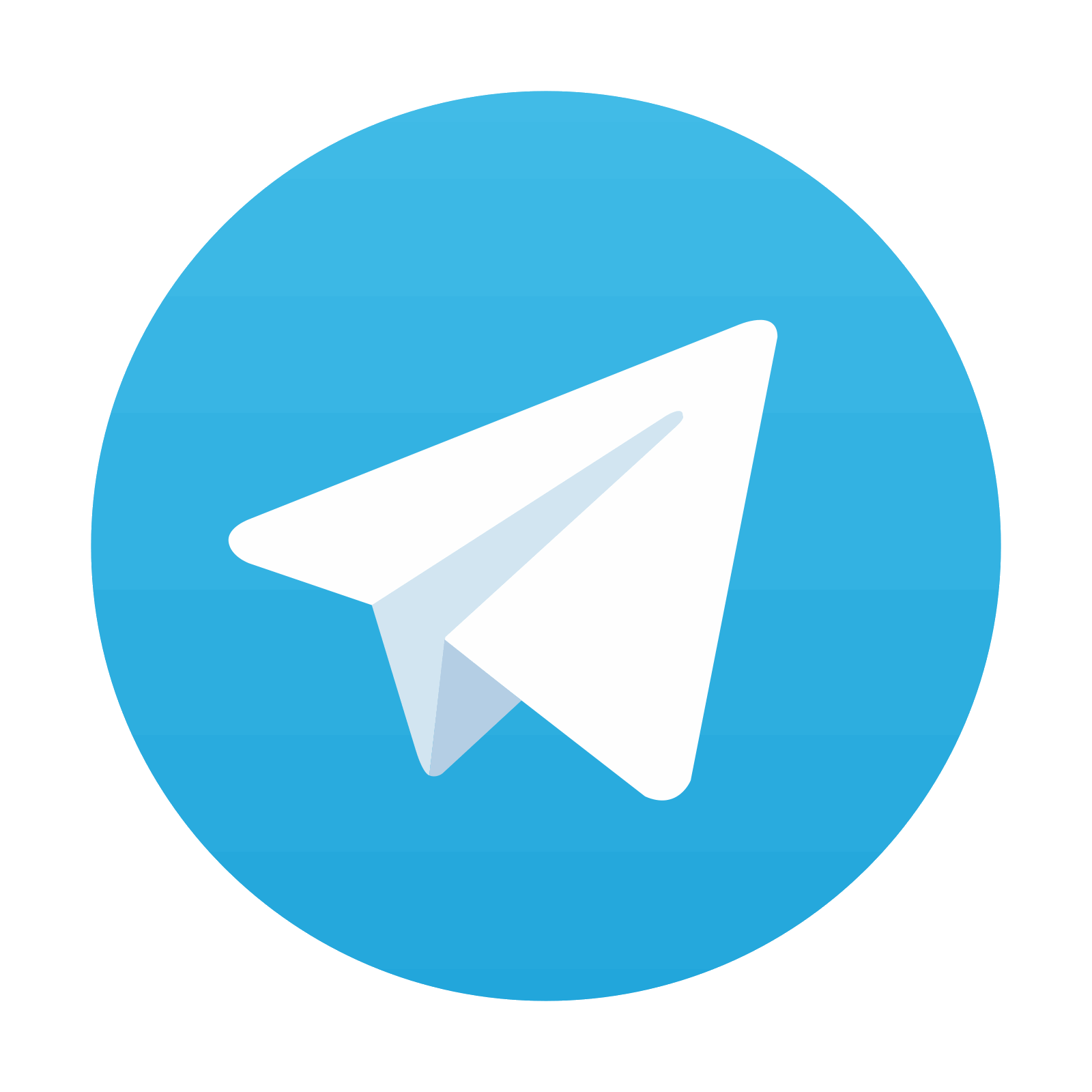
Stay updated, free articles. Join our Telegram channel
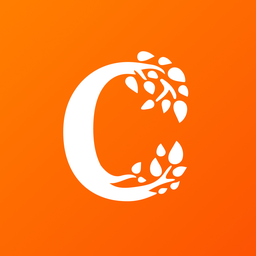
Full access? Get Clinical Tree
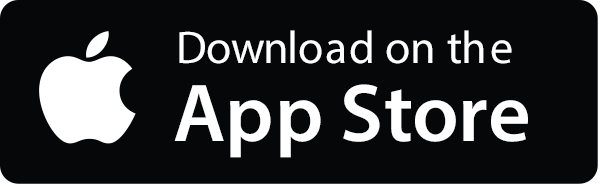
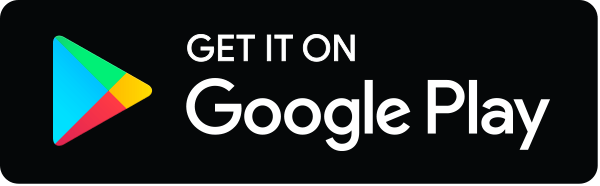