33 Bioabsorbable Stents
One of the major advances in the field of interventional cardiology has been the development of coronary stents. By providing mechanical scaffolding to the vessel, a conventional metallic stent prevents abrupt closure of the vessel, which is a major safety concern with balloon angioplasty. Disruption of the endothelium invariably ensues following angioplasty or stenting and in most cases, approximately 12 weeks are needed for complete re-endothelialization and intimal healing.1 Although stents have significantly reduced acute vessel closure compared with angioplasty, there are several drawbacks. Besides leaving a permanent implant, stenting in the long term may also prevent favorable arterial remodeling.2 Stenting of long lesions, as commonly performed now, may preclude future surgical or percutaneous revascularization, when needed, and may interfere with image interpretations of MRA and CTA because of metallic artifacts. As conventional metallic stents provide stimuli for smooth muscle cell proliferation, neointimal hyperplasia leading to in-stent re-stenosis occurs in up to 30% of patients at 6 months.3–5 Drug-eluting stents (DESs), on the platform of metallic stents, markedly reduce the rate of in-stent re-stenosis by eluting anti-proliferative drugs such as rapamycin, everolimus, zotarolimus, and paclitaxel.6,7 Though DESs have significantly overcome the hurdles of in-stent re-stenosis and have reduced the rate of revascularization, they may cause an increase in stent thrombosis, both early and late, because of either long-term ongoing inflammation or other factors.8–10 Combination anti-platelet therapy with aspirin and clopidogrel is therefore recommended for at least 12 months after a DES to minimize the risks of stent thrombosis.11,12 A significant step forward in stent technology is the development of biodegradable or bioabsorbable stents, which may eliminate some of the concerns and drawbacks of traditional metallic stents. Bioabsorbable stents are made up of materials capable of gradual degradation in the body and thus leave no residual implant within the arteries. A bioabsorbable stent should provide adequate scaffolding for a clinically relevant period (ideally about 6 months) and then disappear. This avoids the potential disadvantages of a permanent metallic implant, may reduce or elimante stent thrombosis stent thrombosis, makes future imaging with CTA or MRA possible, facilitates re-intervention, and may restore vasomotion and prevent side branch obstruction by jailing from metallic struts.13 An effective bioabsorbable stent will perform acutely like a current-generation metallic DES with the same or better long-term safety profile. Only after these criteria have been met will the advantages of bioabsorption encourage the widespread adoption of bioabsorbable stents. A successful design is a combination of numerous factors, including material selection, absorption profile, drug efficacy, deliverability of the stent system, and acute mechanical performance of the stent. Table 33-1 lists the advantages and disadvantages of bioabsorbable stents compared with traditional metallic stents.
TABLE 33-1 Advantages and Disadvantages of Bioabsorbable Stents
Bioabsorbable Materials
Beginning with absorbable sutures in the 1960s, bioabsorbable polymers have been employed in a wide variety of medical devices. Polymers manufactured from lactide and glycolide-based polymers are the most commonly used materials for clinically approved devices. The safety of these polymers, as both vascular and nonvascular device ingredients, has been well demonstrated, with hundreds of devices being approved for human use over the past four decades. The mechanical properties of a polymer are tensile strength, modulus and strain-to-failure affect recoil, expansibility, flexibility, and deliverability of a stent. Thus, a suitable polymer should have high tensile strength, high modulus, and optimal elongation to allow the creation of a low-profile, balloon-expandable stent design. The degradation rate and the degradation products of the polymer affect the duration of vessel support and the degree of tissue reaction. Chemical and thermal properties determine the type of degradation products and the type of processing and sterilization methods that can be used. The characteristics of a suitable polymer for intravascular stenting are listed in Table 33-2; these include optimal mechanical strength, degradation profile, and biocompatibility.14 The bioabsorbable stents currently under investigation do not meet all of the criteria to be considered optimal. The physical properties of a polymer are determined by its hydrophilicity, crystallinity, molecular-weight distribution, and end groups and the presence of residual monomers or additives.15–17 Table 33-3 lists the physical properties of commonly used biodegradable polymers. Polyglycolic acid (PGA), the polymer of glycolic acid, is the simplest linear aliphatic polyester. PGA is highly crystalline with a high melting point and a degradation period of 6 to 12 months. Polylactide (PLA) is the polymer of lactic acid. Lactic acid has two optical isomers—L-lactic acid, the naturally occurring isomer, and D-lactic acid. Poly D,L-lactic acid (PDLLA) contains both D-lactide and L-lactic acid. Poly-L-lactic acid (PLLA) is the homopolymer of L-lactide. The degradation time of PLLA is much slower than that of PDLLA. As PDLLA is amorphous, with low tensile strength, higher elongation time, and rapid degradation rate, it is highly suitable for a drug delivery system. Table 33-4 lists the ideal properties of a polymer suitable for drug elution. In contrast, PLLA is semi-crystalline, with a slower degradation rate and higher tensile strength and thus seems to be ideal for use as a stent. Tissue response to the degradation products depends on the rate of degradation, size and site of the implant, and local tissue microenvironment (pH, acidity, etc.). Lactide-based and glycolide-based polymers undergo degradation by hydrolysis, which leads to the formation of water-soluble, low-molecular-weight components, which are metabolized into carbon dioxide and water. Bulk erosion occurs when the rate at which water penetrates the device exceeds that at which the polymer is converted into water-soluble materials, which results in erosion throughout the device. Strongly hydrophobic polymers undergo mostly surface erosion, a process that occurs when the rate at which water penetrates the device is slower than the rate of conversion of polymer into water-soluble material, which leads to device thinning over time but maintains its bulk integrity.18–20 Figures 33-1 and 33-2 portray the degradation process of bioabsorbable polymers. Limitations of a polymer-based bioabsorbable stent include potential for inflammation and a bulkier profile compared with metallic stents. The search for potential alternatives to bioabsorbable polymers as stent materials has led scientists to investigate the scope of absorbable metals. An ideal absorbable metal stent should have mechanical properties similar to conventional metal stents, have sustained mechanical integrity, have steerable kinetics, induce normal endothelial function with minimal or zero inflammatory or thrombogenic response, and degrade into nontoxic byproducts. Magnesium and iron meet most of these criteria and have been further evaluated. These two metals, even at high doses, were found to have no cytotoxic or genotoxic effects, are devoid of acute systemic toxicity, and are hemocompatible with no chronic toxicity. In general, metallic stents are found to have higher collapse pressure and lesser degree of recoil, thus providing efficient scaffolding.21
TABLE 33-2 Characteristics of an Ideal Bioabsorbable Stent Material
TABLE 33-4 Ideal Characteristics of a Drug-Eluting Bioabsorbable Polymer
Polymer-Based Bioabsorbable Stents
The first biodegradable stent, made of a polymer of poly-L-lactide (PLLA) was developed in the early 1980s by Stack et al and implanted in animal models at Duke University in the 1990s.22 Although this stent could withstand up to 1000 mm Hg of crush pressure, almost twice as much compared with a Palmaz–Schatz stent, could maintain its radial strength for 1 month, and degraded completely by 9 months, further progress in the research of this polymeric stent was somewhat slow. The Duke bioabsorbable stent was the prototype of the PLA stent used in canine femoral arteries and was self-expanding. The long-term degradation of this stent caused little thrombotic response, minimal neointimal hyperplasia, and minimal inflammatory reaction.22 The Kyoto University biodegradable stent, made of polyglycolic acid, developed a few years after the Duke stent, was more thrombogenic when implanted in a canine model.23 The Cleveland clinic–Mayo–Thoraxcenter biodegradable stent consisting of five different biodegradable polymers (PDLLA, polyorthoester, poly-hydroxybutyrate/hydroxyvalerate, polycaprolactone, and polyethelene oxide) exhibited marked inflammatory reaction with neointimal proliferation in porcine coronary arteries.24 This intense local reaction—as demonstrated by a marked inflammatory reaction, neointimal proliferation, medial necrosis, and pseudo-aneurysm formation—was observed in the case of both bioabsorbable and biodurable polymers. Thus, the observed response (at 28 days) was not caused by the formation of degradation products. The inflammatory response was most likely caused by the use of nonsterile implants and a less-than-ideal geometry. When the results obtained in this study are compared with the low-thrombotic and low-inflammatory response obtained with the Duke PLLA stent, it becomes apparent that vessel response is driven by a combination of parameters. An effective bioabsorbable stent must therefore combine an ideal material, adequate sterilization techniques, and an optimal design to achieve desirable results. In a further evaluation of bioabsorbable stents, Lincoff et al demonstrated that the differential degree of local reaction in a porcine coronary injury model was dependent on the size of the polymers, with the lighter PLLA polymers (molecular mass about 80 kiloDaltons [kDa]) being more inflammatory and the heavier polymers (molecular mass about 321 kDa) being less so.25 Yamawaki et al coated the biodegradable stent with an anti-proliferative drug, a tyrosine kinase inhibitor (tranilast), to suppress this local reaction and found that there was a reduced risk of stenosis with the drug–polymer combination.26
Poly L-Lactic Acid Bioabsorbable Stents
Coronary Stents
The collapse pressures of PLLA stents are nearly equal to that of stainless steel metal stents, which makes these polymers suitable for bioabsorbable stents.20 The Igaki–Tamai stent (Igaki Medical Planning Company, Kyoto, Japan), a mono-filamentous, PLLA-based, 183-kDa biodegradable stent, was the first bioabsorbable stent implanted in humans (Fig. 33-3). It has been improved from its earlier versions with a zigzag, helical coiled design that may minimize tissue injury and thereby reduce local tissue reaction and thrombus formation.27–29 A combination of the heat-expandable and balloon-expandable properties of the stent allowed its initial self-expansion in response to heat (transmitted by a delivery balloon inflated with 70oC contrast-water mixture and 50o
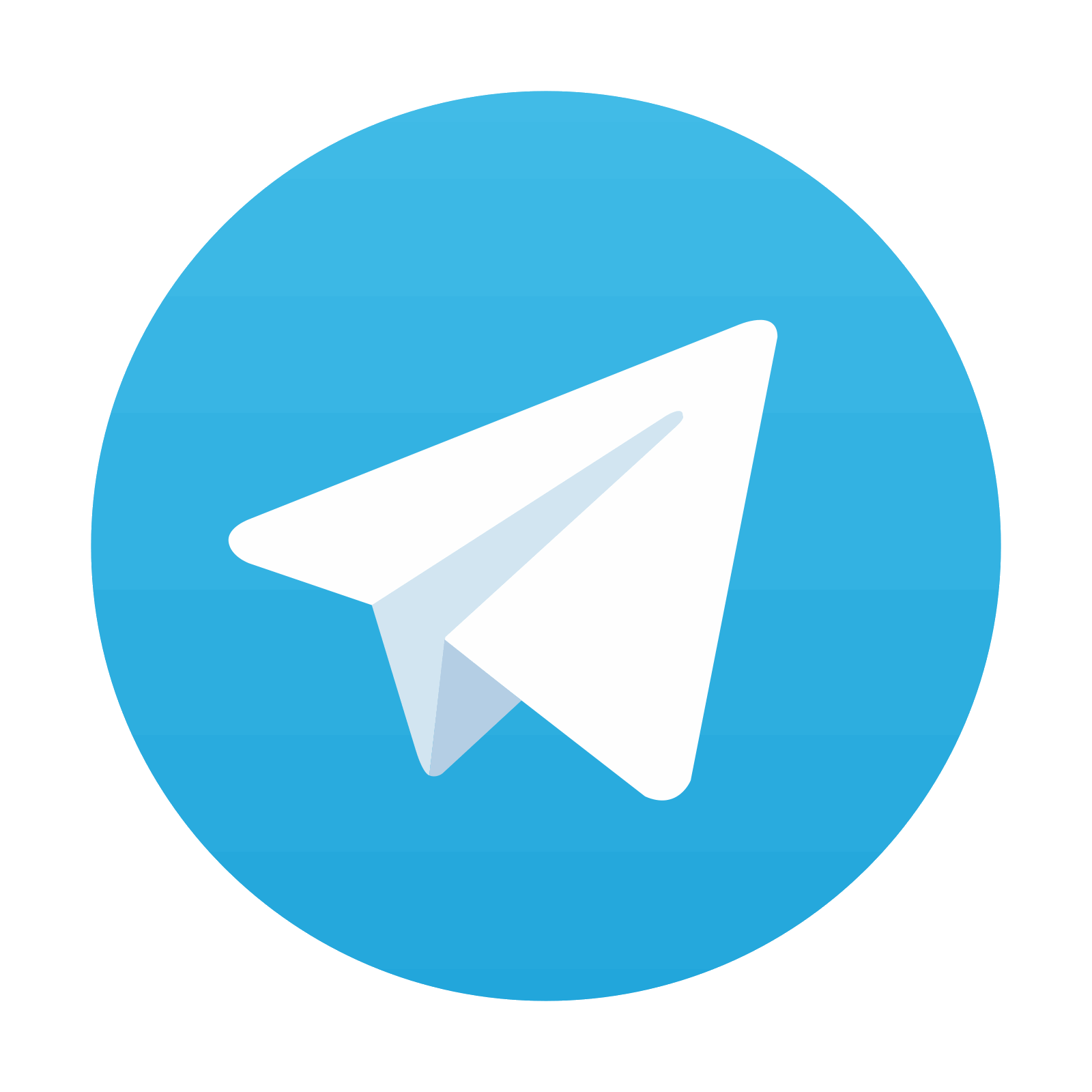
Stay updated, free articles. Join our Telegram channel
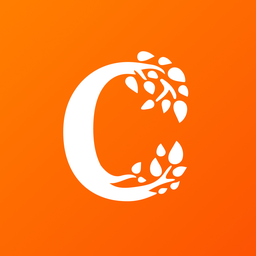
Full access? Get Clinical Tree
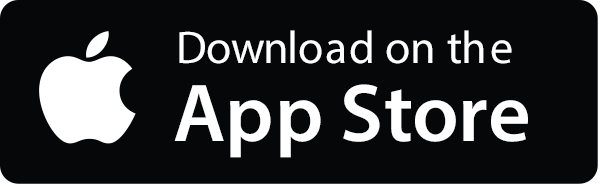
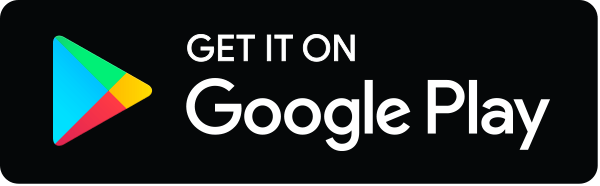