Type of syndrome
Clinical features
Type 1
Acute worsening of heart function leads to acute kidney injury and/or dysfunction
Acute cardiorenal syndrome
Type 2
Chronic abnormalities in heart function result in kidney injury and/or dysfunction
Chronic cardiorenal syndrome
Type 3
Acute worsening in renal function causes heart injury and/or dysfunction
Acute renocardiac syndrome
Type 4
Chronic renal dysfunction induces heart injury and/or dysfunction
Chronic renocardiac syndrome
Type 5
Systemic diseases (diabetes, amyloidosis, systemic lupus erythematous, sepsis) leads to simultaneous injury and/or dysfunction of heart and kidney
Secondary cardiorenal syndromes
It has been estimated that nearly 40 % of patients with acute heart failure also develop acute kidney injury (CRS type 1). Patients with chronic heart failure (HF) demonstrate CKD in 45–64 % of cases (Heywood et al. 2007). Of note, chronic HF and renal failure coexist in the chronic syndromes (types 2 and 4), and it is sometimes hard to distinguish the primary cause of CRS (Ronco et al. 2010). Patients may also move between the subtypes of CRS during the course of their disease (Hause et al. 2010).
In this paragraph, we focus on CRS type 2 and type 4. In the next paragraph, we discuss the derangements in the SNS in patients with various stages of CKD. Next, we review the current literature on [123I]-MIBG SPECT in patients with CKD including the practical application of [123I]-MIBG SPECT in CKD.
20.1.1 Chronic Cardiorenal Syndrome (CRS Type 2)
The prevalence of HF is estimated to be 2–3 % globally and increases with age. The 5-year mortality rate approximates 50 % (Dickstein et al. 2008). At the early stages of heart dysfunction, sympathetic activity increases to preserve circulatory homeostasis. This results in cardiac sympathetic stimulation and has chronotropic (increase in heart rate), inotropic (increase in contractile force), dromotropic (elevated atrioventricular conduction), and bathmotropic (increase in excitability) effects. Sympathetic activation also causes a rise in peripheral vascular resistance, sodium and water retention, and activation of other neurohormonal systems like the renin-angiotensin system (RAS) (Carrió 2001; Henneman et al. 2007; Martins da Silva et al. 2013). In patients with chronic heart failure, the sympathetic activity is up to 50 times higher than in healthy controls (Malpas 2010). The long-term stimulation of SNS has a negative impact on the CV system: the sustained sympathetic overactivity leads to changes in cardiac structure and function and contributes to the development of cardiac hypertrophy, myocyte apoptosis, fibroblastic proliferation, interstitial collagen accumulation, and myocardial fibrosis (Adameova et al. 2009; Martins da Silva et al. 2013).
Of note, the hyperactivity of SNS increases the susceptibility for arrhythmias and, thus, leads to an increased risk of cardiovascular mortality and in particular SCD (Boogers et al. 2011). [123I]-MIBG imaging was also used to predict the outcome of HF (Verberne et al. 2008a). Further, it was found that an abnormal H/M ratio with [123I]-MIBG imaging is an independent predictor of cardiac death, even better than impaired left ventricular ejection fraction (Merlet et al. 1999).
Hypertension and atherosclerosis are major risk factors of extensive morbidity and mortality in patients with CKD. Hypertension occurs in 80 % of patients with advanced CKD (Converse et al. 1992). The etiology of hypertension in CKD is multifactorial with hypervolemia, activation of RAS, and SNS overactivity as its major determinants (Koomans et al. 2004; Blankenstijn 2004; Kotanko 2006).
Furuhashi and Moroi analyzed the mortality rate and cardiac [123I]-MIBG uptake in patients with heart failure in relation to their renal function, excluding dialysis patients (Furuhashi and Moroi 2007). They found that among patients with heart failure and a glomerular filtration rate ≥60 ml/min/1.73 m2, the mortality rate was lower (9 %) than in patients with heart failure and a glomerular filtration rate <60 ml/min/1.73 m2 (19 %). Interestingly, the most powerful predictor of cardiac death in the group with a glomerular filtration rate ≥60 ml/min/1.73 m2 was a delayed H/M ratio higher than 146 %. In contrast, in patients with a glomerular filtration rate <60 ml/min/1.73 m2, the results of [123I]-MIBG myocardial imaging had no effect on the incidence of cardiac death (Furuhashi and Moroi 2007).
20.1.2 Chronic Renocardiac Syndrome (CRS Type 4)
CKD is currently defined by Kidney Disease: Improving Global Outcomes (KDIGO) as “abnormalities of kidney structure or function, present for >3 months, with implications for health” (KDIGO CKD guideline 2012). CKD is classified based on cause, GFR category, and albuminuria category (KDIGO CKD guideline 2012). CKD is a significant problem, as, since 1980, in Central Europe, the number of patients treated with renal replacement therapy has doubled every decade (Rutkowski 2006). This phenomenon is associated with a higher prevalence of risk factors for CKD such as hypertension and diabetes within the general population. Besides the ‘traditional’ risk factors for CKD, a kidney-specific risk factor is hyperactivity of the SNS (Blankenstijn et al. 2011).
With the onset of CKD, the likelihood of CV complications increases in both symptomatic and asymptomatic heart failure patients (Dries et al. 2000; Van Domburg et al. 2008; Schrier 2006; Caglar et al. 2006; Gansevoort et al. 2013). Dysfunction of the cardiovascular system is the cause of at least 40 % of deaths in patients with ESRD, of which one-fourth is sudden cardiac death (Zipes et al. 2006; McMahon 2003; Herzog 2007).
The main role of the kidney is the regulation of extracellular fluid volume. Renal failure is characterized by increased sodium and water retention. The subsequent increase in extracellular fluid volume leads to hypertension and excessive cardiac preload and contributes to heart enlargement and dysfunction. These changes finally lead to mitral valve insufficiency and pulmonary hypertension, as well as to right and left ventricular failure (Schrier 2006). CKD is also often associated with anemia, which, together with hypertension and increased levels of catecholamines, predisposes for the development of left ventricular hypertrophy. Systemic inflammatory reactions may also contribute to the development of atherosclerosis (Ross 1999). Moreover, increased serum levels of PTH and phosphorus are associated with increased vascular calcifications and calcification of the heart valves (Schrier 2006) (Fig. 20.1).
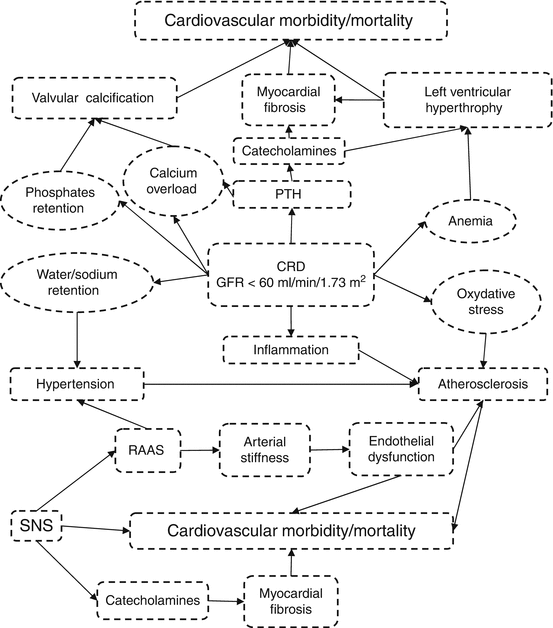
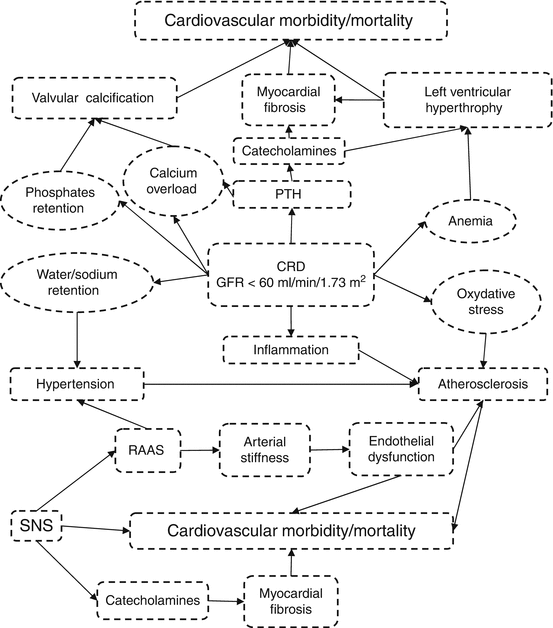
Fig. 20.1
Metabolic interactions in CKD
20.2 Hyperactivity of the Sympathetic Nervous System in CKD
As mentioned earlier, the development of chronic kidney disease is closely related to increased activation of the SNS. Kidney injury or renal ischemia is the trigger mechanism of activation of SNS and RAS (Vink and Blankenstijn 2012; Vink et al. 2013). Decreased renal oxygen supply induces adenosine release, which stimulates the rise in blood pressure, probably by afferent renal nerve activation (Katholi et al. 1984). Hyperactivity of renal SNS and RAS affects renal and vascular function (DiBona 2000, 2001). Somatic afferent impulses arising from the failing kidney cause an increase in central sympathetic drive. The kidney not only generates afferent sympathetic activity but also receives efferent sympathetic signals (Sobotka et al. 2011; Vink and Blankenstijn 2012; Vink et al. 2013). Efferent stimulation triggers a cascade of actions in the kidney. First, renal vasoconstriction leads to a reduction in renal blood flow and glomerular filtration rate. Renin is released and this stimulates angiotensin II production. This process augments the direct activation of RAS by kidney ischemia (Reid 1992). Decreases in brain nitric oxide levels and increased oxidative stress, which is characteristic of CKD, may further sensitize various organs in CRS for the damaging action of sympathetic overactivity.
The first indirect evidence that hyperactivity of SNS originates from the kidney was provided more than 40 years ago in ESRD patients, in whom hypertension and peripheral resistance were treated by bilateral nephrectomy (Kim et al. 1972). The direct evidence of hyperactivity of SNS in CKD patients was provided by muscle sympathetic nerve activity (MSNA) in microneuronography (Converse et al. 1992). In these patients, bilateral nephrectomy stopped the afferent stimulation and resulted in normalization of sympathetic activity (Converse et al. 1992; Hausberg et al. 2002). In an animal model, dorsal rhizotomy prevented elevation of blood pressure (Campese 1997). Likewise, renal denervation prevented the rise of blood pressure in animals with acute renal injury (Ye et al. 1997).
The second method of direct evaluation of cardiac SNS (CSNS) activity is myocardial scintigraphy by use of [123I]-MIBG. It has been found that an extensive release of norepinephrine (NE) into the synaptic cleft reduces the production of membrane NE transporter (NET) protein and NE presynaptic reuptake (Caldwell et al. 2008). [123I]-MIBG stores in the postganglionic presynaptic endings of sympathetic neuron vesicles by the same mechanism as NE and is mainly taken up by neuronal uptake-1 in membrane NET. To a lesser extent, it is taken up by a nonneuronal mechanism: a carrier-facilitated process (uptake-2) and diffusion (Henneman et al. 2008). The degree of the heart [123I]-MIBG uptake, therefore, reflects the presynaptic tone of the CSNS.
The first observations of enhanced [123I]-MIBG myocardial clearance in hemodialysis patients were published in 1995 by Kurata et al. They noticed that [123I]-MIBG clearance was particularly rapid in hemodialysis patients that had coexisting dysfunction or hypertrophy of the left ventricle (Kurata et al. 1995). Their observations were confirmed in subsequent studies (Miyanaga et al. 1996; Kurata et al. 2000). Decreased neuronal uptake and increased myocardial clearance of [123I]-MIBG in patients undergoing dialysis suggest augmented sympathetic nerve discharge due to its prolonged activation.
Diminution of excessive sympathetic activity by elimination of the renal signal could be an attractive therapeutic option. Recently, in patients with resistant hypertension, selective denervation (efferent sympathetic and afferent sensory fibers) of the kidney can be performed using endovascular radiofrequency ablation (Ahmed et al. 2012; Mahfoud and Böhm 2010). This procedure is associated with a significant fall in blood pressure. Whether renal denervation also reduces the hypertension-associated increased risk of cardiovascular events is presently unknown.
The reduction of sympathetic activity in dialysis patients could also be accomplished by increasing the frequency of the hemodialysis sessions, most likely by less fluid fluctuations (Zilch et al. 2007). Significantly lower risk of overall mortality and cardiovascular mortality in hemodialysis patient could be achieved by cold hemodialysis (Hsu et al. 2012). This procedure can also reduce the dialysis-induced LV regional wall motion abnormalities (RWMA) and in this way may improve hemodynamic stability during hemodialysis (Selby et al. 2006; Selby and McIntyre 2006).
Furthermore, inhibitors of the RAS also reduce sympathetic overactivity (Blankenstijn et al. 2011). Reduction of RAS and SNS hyperactivity are the main goals of therapy with β-blockers and angiotensin-converting enzyme inhibitors (ACEi). [123I]-MIBG imaging was applied to assess the effects of these treatments – cardiac [123I]-MIBG uptake improved after therapy with β-blockers (Agostini et al. 2000) and ACEi (Takeishi et al. 1997). Kasama et al. studied a group of 30 patients with dilated cardiomyopathy (DCM) before and 6 months after standard carvedilol therapy. The authors concluded that the scintigraphic parameters (H/M ratio, WR) as well echocardiographic findings (LVEF) were improved in the study group after long-term β-blocker therapy (Kasama et al. 2007). Takeishi et al. observed H/M improvement and decrease of WR in 19 NYHA class II–III patients treated by enalapril (Takeishi et al. 1997). 123I-MIBG imaging was able to predict the occurrence of ventricular arrhythmias. Boogers et al. performed 123I-MIBG SPECT study in 116 patients with CHF before implantable cardioverter-defibrillator (ICD) implantation. The late 123I-MIBG images were independent predictive factor of appropriate ICD therapy (Boogers et al. 2010). In the ADMIRE-HF study, Jacobson et al. found that arrhythmic events were common in patients with H/M ratio <1.6 (10.4 %) compared with those with H/M ratio >1.6 (3.5 %) (Jacobson et al. 2010).
20.2.1 Intradialytic Hypotension
Intradialytic hypotension (IDH) is one of the most frequent complications of hemodialysis treatment. It is estimated to occur in 10–50 % of hemodialysis sessions (Daugirdas 2001; Orofino et al. 1990; Palmer and Henrich 2008). Dialysis hypotension can lead to serious vascular complications such as cerebral infarction and mesenteric ischemia (Schreiber 2001a, b; John et al. 2000). It is increasingly recognized that IDH may also precipitate myocardial ischemia (Zuidema and Dellsperger 2012; McIntyre 2009). This will be discussed in the next paragraph. The pathogenesis of IDH is complex, but hypovolemia as a result of an imbalance between the ultrafiltration rate and the plasma refilling rate is generally believed to be the initiating factor (Koomans and Blankestijn 1995; van der Sande et al. 2000). Higher ultrafiltration rates (over 13 ml/h/kg) are not only associated with a greater risk of IDH but also with a significantly higher all-cause and CV mortality (Flythe et al. 2011). Importantly, frank dialysis hypotension only occurs when the CV compensatory mechanisms can no longer compensate for the reduction of blood volume (Daugirdas 2001; Palmer and Henrich 2008). Patients with autonomic insufficiency are at increased risk of IDH since an adequate cardiovascular response to hypovolemia depends on intact autonomic function (Sato et al. 2001). However, the relationship between autonomic insufficiency and the risk of IDH is not fully elucidated with some studies reporting a significant association between worse autonomic function and a higher susceptibility for IDH (Kersh et al. 1974; Enzmann et al. 1995; Sato et al. 2001), whereas other studies did not find such an association (Naik et al. 1981; Nakashima et al. 1987; Straver et al. 1998; Sapoznikov et al. 2010). Sato et al. assessed autonomic function by means of myocardial [123I]-MIBG uptake and assessment of early and late H/M ratio in four groups of patients: diabetic patients with and without frequent IDH and nondiabetic patients with and without frequent IDH. Patients with frequent IDH had more severe autonomic insufficiency (the lowest H/M ratio) compared with patients without IDH, while the coexistence of diabetes enhanced this abnormality (Sato et al. 2001). The authors concluded that in patients with IDH, special attention should be given not only to dry weight but also to autonomic insufficiency. Notably, the treatment of IDH often involves reducing or withdrawing ultrafiltration, which may cause volume overload and premature stop of the dialysis session and, if repetitive, inadequate removal of uremic toxins and fluid (Palmer and Henrich 2008). In such patients, longer and/or more frequent hemodialysis sessions may be warranted to achieve adequate fluid control and removal of uremic toxins.
20.2.2 Hemodialysis-Induced Reductions in Cardiac Perfusion and Function
Although hemodialysis is a lifesaving procedure, recent studies have shown that conventional hemodialysis may have acute adverse effects on cardiac perfusion and function. McIntyre et al. (2008) studied the acute effect of hemodialysis on cardiac perfusion and left ventricular function using serial intradialytic positron emission tomography (PET) with radiolabeled water (H2[15O]) and echocardiography in four patients (three with diabetes) without significant coronary disease on coronary angiography. They found that global myocardial blood flow (MBF) in before dialysis was in the normal range, whereas during hemodialysis significantly fell down. All four patients developed RWMA of the left ventricle. A reduction in MBF of >30 % from baseline was significantly associated with the development of RWMA. Interestingly, the fall in segmental MBF was significantly higher in regions that developed RWMA compared with regions that preserved normal function. At 30 min post-hemodialysis, MBF was partially recovered and most, but not all, RWMA had disappeared. Dasselaar (2009) confirmed that an uncomplicated hemodialysis procedure was associated with a significant reduction of MBF. In that study, the myocardial perfusion was evaluated by PET technique by use of radiolabeled ammonia ([13N]NH3) – before, in the beginning and in the end of hemodialysis session. Two of seven patients developed RWMA, and the fall in myocardial blood flow was greater in segments that developed RWMA in comparison with regions that preserved normal left ventricular function. Together, these results strongly suggest that hemodialysis is capable of inducing myocardial ischemia and cardiac stunning.
Presently, the pathogenesis of hemodialysis-induced left ventricular dysfunction is unknown. Burton et al. (2009) identified diabetes, lower albumin levels, a greater reduction in systolic blood pressure, and a higher ultrafiltration volume as risk factors for the development of hemodialysis-induced RWMA. The finding of a relationship between higher ultrafiltration volumes and hemodialysis-induced cardiac dysfunction was not found in a recent study by Assa et al. (2012a). In the study of Dasselaar et al. (2009), MBF fell significantly already early during hemodialysis when ultrafiltration volume was negligible. These studies suggest that not only hypovolemia but also other dialysis-related factors are involved in the pathogenesis of hemodialysis-induced regional left ventricular dysfunction.
Hemodialysis-induced reductions in MBF may contribute to the high mortality rates among hemodialysis patients (McIntyre 2009; Dasselaar et al. 2009). First, hemodialysis-induced cardiac ischemia may trigger arrhythmias. Indeed, the risk of sudden cardiac death is increased during and after hemodialysis session (Bleyer et al. 2006). Cardiac arrhythmias were significantly more frequent in patients with hemodialysis-induced cardiac dysfunction (Burton et al. 2008). Second, repetitive hemodialysis-induced regional ischemia may also lead to cumulative left ventricular dysfunction and eventually result in heart failure, a highly prevalent condition in hemodialysis patients. Burton et al. have shown that patients who develop RWMA during hemodialysis have a faster deterioration of left ventricular function during 1 year of follow-up in comparison with patients who do not develop hemodialysis-induced cardiac dysfunction (Burton et al. 2009).
The autonomic nervous system has an important role in the regulation of MBF. At present, however, cardiac SNS has not been compared between patients with and without cardiac ischemia during hemodialysis.
20.2.3 Hemodialysis Versus Peritoneal Dialysis
Although the nature of peritoneal dialysis and hemodialysis (HD) are essentially different, the cardiovascular outcome of both methods of RRT is comparable and unfortunately very bleak (Sato et al. 2001; Vonesh et al. 2006). However, the prevalence of myocardial stunning is substantially lower in peritoneal dialysis patients compared to HD patients (Selby and McIntyre 2011). Moreover, although hemodynamic instability can occur with peritoneal dialysis (McIntyre 2011), it is much more frequent with hemodialysis treatment than with peritoneal dialysis treatment. This indicates that other factors than myocardial stunning and hemodynamic instability must play a role in the high all-cause and cardiovascular mortality in peritoneal dialysis patients. Several studies have demonstrated reduced heart rate variability in patients with end-stage disease. In one of these studies, a similar depression in autonomic control was demonstrated in hemodialysis (n = 8) and peritoneal dialysis patients (n = 7) (Axelrod et al. 1987).
20.2.4 Influence of Renal Replacement Therapy on Cardiac Adrenergic Function
Kutata et al. reported that autonomic function normalized after renal transplantation (Kurata et al. 2004). They observed a reduction of [123I]-MIBG myocardial washout rate (WR), from 46 ± 21 % before transplantation to 20 ± 22 % after transplantation. Delayed H/M ratio also increased from 1.74 ± 0.39 before renal transplantation to 2.06 ± 0.39 after this procedure (p = 0.006). Similar improvements were observed in children after renal transplantation (Parisotto et al. 2008). In that study, subjects were divided according to the following treatment strategies: conservative treatment, peritoneal dialysis, HD, and renal transplantation. The authors concluded that children undergoing dialysis had various sympathetic abnormalities, related to renal impairment, in the absence of major cardiovascular comorbidities. According to this study, cardiac adrenergic indices, like H/M ratios or WR, normalized after renal transplant, parallel to improvement of graft function, suggesting CSNS recovery (Parisotto et al. 2008).
The uremia-related toxins cannot be completely cleared, even in very effective dialysis. The efficacy of dialysis can be assessed by Kt/V, an index of fractional urea clearance. The adequacy of HD is a highly important factor in the improvement of CSNS function (Laaksonen et al. 2000). This group observed that hemodialysis patients with a Kt/V that was lower than 0.85 showed a progressive deterioration of the CSNS, whereas patients with a Kt/V higher than 1.2 had improved autonomic function, as measured by heart rate variability (HRV) (Laaksonen et al. 2000). In this respect, it is interesting that patients on overnight dialysis sessions showed an amelioration of common sympathetic uremia-related sleep breathing disorders: Chan et al. noticed that augmentation of dialysis dose and frequency in nocturnal dialysis session reduced sleep-related hypoxemia and normalized sympathetic drive as well as the heart rate variability (Chan et al. 2004).
20.3 Technical Aspects of Cardiac [123I]-MIBG
The hyperactive cardiac sympathetic system is characterized by a decrease in [123I]-MIBG uptake and an increase in myocardial washout of this radiotracer. The activity of CSNS can be assessed visually and semiquantitatively. The visual evaluation of regional [123I]-MIBG myocardial distribution is performed by SPECT technique (Fig. 20.2) as well as planar scintigraphy, also in patients with kidney diseases (Fig. 20.3).