Chapter 92
Autogenous Grafts
Scott A. Berceli
Despite ongoing attempts to develop prosthetic or bioengineered materials for bypass grafting in the lower extremities, autologous vein graft remains the conduit of choice for infrainguinal revascularization. Though it was sporadically used for repair of popliteal aneurysms in the early 20th century, the first report of the routine use of autogenous vein for the treatment of occlusive arterial disease was provided in 1944 by Dos Santos, who used segments of vein as patch material after superficial femoral artery endarterectomy.1 This was followed several years later by the report of Kunlin, who described the use of reversed saphenous vein grafts for the treatment of focal lesions within the superficial femoral artery.2 Based on these initial reports, expanded use of the saphenous vein for both endarterectomy and grafting was attempted, but creation of the anastomoses with the standard surgical techniques of the day proved difficult. With long-term patency negatively influenced by a high rate of anastomotic stricture and an inability to replicate the promising results of Kunlin, the majority of practitioners in the 1940s and 1950s used arterial homografts or the newly developed prosthetic grafts for infrainguinal arterial reconstruction. Reinvigorating the use of autogenous vein, however, was a small group of surgeons who traveled to Europe to observe Kunlin’s vein grafting procedures.3 Characterized by a spatulated end-to-side anastomosis using a small-caliber running silk suture, these meticulous techniques allowed Linton, Darling, and others to duplicate the reported success with autogenous vein grafting.4
In the 1960s, wider interest in the use of autogenous vein was fueled by the observation that acceptable long-term patency of prosthetic bypass grafts could be achieved only with distal anastomoses placed in the above-knee position.5 Treatment of critical limb ischemia in patients with compromised outflow via femorotibial bypass was a natural extension for autogenous vein grafting, with the first published report by Dale in 1963.6 Although initial published reports of the use of saphenous vein for tibial bypass grafts suggested it to be notably inferior to femoropopliteal grafts,7,8 further refinements in the techniques have proved the durability of tibial as well as inframalleolar bypass grafting with autologous vein.9 Along with these technical improvements in the 1980s came increasing experience with alternative autogenous vein sources (i.e., arm, small saphenous, and femoral veins),10 thereby expanding the potential for autogenous tissue reconstruction to 95% of patients undergoing infrainguinal bypass.11
Histology
Analogous to other vascular structures, veins are composed of three layers—the tunicae intima, media, and adventitia (Fig. 92-1). The intima consists of a continuous monolayer of cuboidal endothelial cells supported by a subendothelial layer of loose connective tissue. In contrast to the arterial endothelium, where cells are tightly packed and highly aligned in the direction of flow, venous endothelial cells are cuboidal with poorly developed interendothelial tight junctions.12,13 The resulting enhanced permeability to blood solutes, in conjunction with the underlying physiologic differences between arterial and venous endothelium, is thought to be among the underlying mechanisms for the accelerated intimal hyperplasia characteristic of vein grafts.14 The intima is separated from the media by a poorly developed internal elastic lamina with large fenestrae that allow diffusion of substances into the wall. The media is composed of vascular smooth muscle cells and extracellular matrix, with type I collagen being the dominant component of this layer. Unlike arteries, which function in a pulsatile pressure environment and correspondingly have high elastin content, veins are exposed to small excursions in pressure and demonstrate only sparse elastin fragments. Transposition of a vein into the arterial circulation induces a marked increase in intraluminal wall tension that greatly exceeds the dynamic range of the elastic elements and therefore leads to a rigid graft with substantial compliance mismatch in comparison to the adjacent artery.15
Figure 92-1 Cross-sectional histology of the normal saphenous vein. The intima (I) is composed of a single layer of endothelial cells and a thin basement membrane. The media (M) consists of smooth muscle cells surrounded by a tightly packed extracellular matrix. The adventitia (A) is a loose network of collagen-dominated extracellular matrix with sporadic inflammatory cells and fibroblasts.
External to a poorly developed external elastic lamina is the adventitia, a loose connection of extracellular matrix with a sparse vasa vasorum. Though traditionally thought to have limited influence on biologic function of the vein, evolving evidence suggests that remodeling of an implanted vein graft is very much dependent on adaptation within the adventitia. Recruitment of myofibroblasts, production of cytokines, and the influx of inflammatory cells into the adventitia are thought to have an important influence on the biology of the early vein graft.
Superficial extremity veins are prone to the development of a range of pathologies such as varicose degeneration, thrombosis with luminal recanalization, and phlebitis with intramural calcification. Limitations in available autogenous conduit frequently require these “compromised” vein segments to be included as conduit in a revascularization procedure. Histologic evaluation of harvested vein segments, coupled with long-term clinical follow-up, has demonstrated that grafts with low endothelial cell coverage, stenosis of the lumen, and thick walls are at an increased risk for early graft failure.16 Grafts with subendothelial spindle-shaped cells, which demonstrate a proliferative smooth muscle phenotype on electron microscopic examination, and calcification within the wall at the time of graft implantation are notably prone to the development of aggressive hyperplastic lesions.17 Unfortunately, preoperative ultrasound is unable to reliably identify many of these abnormalities.18
Anatomy
Lower Extremity Veins
The veins of the lower extremities are classified into three general groups: the deep, superficial, and perforating systems. The deep veins are encompassed by thick fascia and are contained within the muscular compartment of the leg. Superficial veins are bounded deeply by the muscular fascia and superficially by the dermis. Short veins piercing through the fascia and running between the superficial and deep systems are the perforating veins. Increasing interest in accurate description of the venous structures in the leg and confusion between the clinical and anatomic terminology used in naming them have prompted a review and standardization of the nomenclature. The following descriptions are based on this consensus terminology,19,20 with reference to the older nomenclature as appropriate.
The great saphenous vein (formerly called the greater or long saphenous vein) begins just anterior to the medial malleolus, crosses the tibia, traverses medial to the knee, and ascends in the medial-posterior aspect of the thigh to the groin. Duplicated systems within the great saphenous vein are relatively common and occur at a 25% and 8% incidence in the calf and thigh, respectively.21 The accessory great saphenous veins run parallel to the main trunk within the thigh and leg, in an anterior or posterior location. Although these veins are at times mistaken for the great saphenous vein during surgical exposure, they are usually of inadequate diameter for use in bypass grafting. The saphenous veins, but not their tributaries, are covered by a fibrous sheath termed the saphenous fascia. Less well developed than the deep fascia, this fascia, in combination with the deeper muscular fascia, forms a subcompartment that extends along the length of the lower extremity. Recognition of this fascia can be useful in localizing the great saphenous vein during surgical dissection. In the proximal portion of the thigh, the great saphenous vein receives one or two large tributaries from the anterior or posterior accessory great saphenous vein (or both) and enters the fossa ovalis approximately 4 cm inferior and lateral to the pubic tubercle, where it joins with the superficial circumflex iliac, superficial epigastric, and external pudendal veins to create the confluence of superficial inguinal veins (formerly called the saphenofemoral junction) on the anterior surface of the common femoral vein.
The small saphenous vein (formerly called the lesser or short saphenous vein) ascends lateral to the Achilles tendon in the calf. It runs in the subcutaneous adipose tissue in the lower two thirds of the calf, pierces the muscular fascia, and courses between the medial and lateral heads of the gastrocnemius. The small saphenous vein usually joins the popliteal vein in the popliteal fossa about 5 cm proximal to the knee crease. Rarely, the small saphenous vein ends high, either emptying into the femoral vein or running superficially to the posteromedial aspect of the thigh to join the great saphenous vein.
Particularly useful in arterial reconstructions within infected fields, the femoral vein (formerly called the superficial femoral vein) has increasingly become a potential source of autogenous conduit. The femoral vein originates from the popliteal vein at the upper margin of the popliteal fossa and courses in the femoral canal. The femoral vein is lateral to the femoral artery in the distal part of the thigh but crosses under and runs medial to the femoral artery in the proximal portion of the leg before joining the deep femoral (profunda femoris) veins approximately 9 cm below the inguinal ligament. During harvest of the femoral-popliteal vein segment for use as an autogenous graft, preservation of the junction of the profunda femoris vein with the common femoral vein is critical for maintaining adequate venous outflow from the limb.22,23
Upper Extremity Veins
Veins of the upper extremity are also divided into superficial and deep groups, although direction of flow from superficial to deep is not as distinct as in the lower extremity. The cephalic vein, which originates on the posterolateral aspect of the wrist, gradually swings across the lateral portion of the forearm, ascends lateral to the biceps muscle into the deltopectoral groove, and passes through the clavipectoral fascia to join the axillary vein. The basilic vein swings across the posteromedial side of the forearm, passes lateral to the antecubital fossa, and ascends medially in the arm, where it joins the brachial veins to become the axillary vein. The median cubital vein starts at the apex of the antecubital fossa as a branch of the cephalic vein and ascends medially to enter the basilic vein.
Approximately 10 cm proximal to the antecubital fossa, the basilic vein passes through the deep fascia to reside along the medial side of the brachial artery. Harvest of the basilic vein proximal to this location requires longitudinal division of this fascia and ligation of multiple communicating branches coursing between the basilic and brachial veins. During this portion of the dissection, the medial cutaneous nerve of the forearm is often first identified as it travels anterior to the basilic vein. Injury to this nerve can lead to paresthesias along the medial portion of the forearm.
Vein Graft Preparation
Preoperative Vein Mapping
As surgeons have increased the complexity of lower extremity reconstruction and become more aggressive with repeat revascularization after graft failure, assessment of available autogenous conduit has evolved into an essential component of preoperative planning. Initial attempts to evaluate available conduit centered around the use of routine preoperative saphenous venography, with information obtained from these studies influencing the operative approach in up to 30% of patients.24 Although useful in defining the anatomy and dimensions of the greater saphenous system, broad application of this technique was limited by its requirement for percutaneous access, frequent underestimation of conduit diameter, and the small but worrisome risks of nephrotoxicity or chemically induced thrombophlebitis.
With refinements in ultrasound instrumentation and the increasing availability of skilled technologists, investigations into the merits of B-mode imaging for preoperative conduit evaluation were initiated.25–28 By providing the ability to determine vein size and quality in a physiologic environment and facilitate operative exposure, duplex vein mapping generated significant enthusiasm. Early reports were mixed, however, with concern that duplicate saphenous systems were frequently not identified and diameters measured on B-mode imaging were not representative of in vivo graft geometries.25 Although the former issue has essentially been solved by increased experience of vascular technologists and an understanding of anatomic variants, difficulties with direct correlation of preoperative mapping and graft size remain. Among the important issues in minimizing this variability is the development of a standard preoperative evaluation protocol.
Warming the examination room, placing warmed blankets on the extremities, and using prewarmed ultrasonic gel minimize peripheral venous vasoconstriction. Imaging with a high-frequency probe (≥8 MHz) provides maximum resolution with 0.3-mm point-to-point discrimination. Veins are assessed in cross-section to evaluate compressibility, wall thickening, and the presence of intraluminal echoes, and sites of thrombus, intraluminal webs, and sclerotic walls are noted. Vein wall abnormalities have been described in 12% of potential conduits, with preoperative duplex evaluation correctly identifying 62% of these diseased segments.29 To assess the great saphenous vein, patients are placed in a modified reversed Trendelenburg position and images collected for measurement of lumen diameter at six locations along the length of the limb. Evaluation of the small saphenous vein is performed with the patient in a prone position and the diameter measured at three locations within the calf. If either of the saphenous veins is of good quality but inadequate size, the patient is placed in a standing position and diameter measurements are repeated. Alternatively, a tourniquet may be placed on the proximal part of the thigh to facilitate this reassessment. For segments identified to be of adequate size and quality for bypass grafting, the overlying skin is marked with indelible ink. Routine evaluation of the femoral vein may also be warranted depending on local clinical practice. In addition to lumen diameter, examination should include a thorough assessment for deep venous thrombosis and valvular incompetence. Use of standardized reference zones facilitates the reporting of relevant information, with zones 2, 3, and 4 corresponding to the proximal, middle, and distal portions of the thigh and zones 5, 6, and 7 corresponding to the proximal, middle, and distal portions of the calf, respectively.30 Evaluation of the upper extremity cephalic and basilic veins should follow a similar protocol. An upper arm tourniquet may be used selectively or routinely to facilitate these measurements. Reference zones have also been established for the upper extremity, where zones 3, 4, and 5 correspond to the proximal, middle, and distal portions of the arm and zones 6, 7, and 8 correspond to the proximal, middle, and distal portions of the forearm, respectively.30 Measurements of upper extremity vein diameter seem even more variable than those of the lower extremity. Although the underlying etiology is open to speculation, this variability should be recognized, and repeat testing of borderline upper extremity veins may prove valuable in preoperative planning.
Despite multiple attempts to define acceptable conduit size, no clear consensus has emerged. Smaller-diameter veins have repeatedly been shown to negatively influence long-term patency,31,32 and a minimum suitable diameter ranging between 2.0 and 3.0 mm has been proposed.33 Among the confounding issues is the variable effect of the mapping technique on approaching an accurate in vivo graft diameter. These variations are nonlinear and in part dependent on the initial size of the conduit; whereas a 2.5-mm vein increases 27% in diameter when placed in the arterial circulation, a 6-mm vein increases only 8%.27 In the absence of a universally accepted vein mapping protocol, absolute delineation of a minimum acceptable vein size is problematic, and development of size criteria within an institutional practice is most appropriate.
Vein Handling Considerations
Research over the last decade has uncovered the importance of the endothelium in vascular injury and repair. Previously thought to be a passive monolayer with predominantly barrier functions, endothelial cells have been identified as central mediators in the maintenance of vascular homeostasis. Advanced by the work of Ross and his development of the response-to-injury hypothesis,34–36 loss of endothelial integrity induces platelet deposition and elaboration of growth factors. Coupled with recruitment of inflammatory mediators, these growth factors stimulate smooth muscle proliferation and matrix deposition, which lead to the formation of an occlusive arterial lesion. Analogous mechanisms are thought to be fundamental in the pathologic remodeling of vein grafts, with early endothelial injury among the initiating events in the development of intimal hyperplasia.37 Accordingly, maintenance of an intact endothelial monolayer during vein graft harvest is thought to provide improved long-term patency of autogenous vein grafts. Work by LoGerfo38 and others39 in a canine model supports this contention by demonstrating that smooth muscle cells are maintained in a contractile (nonproliferative) phenotype and that leukocyte infiltration is reduced when endothelial injury is avoided. Analogous work by Conte’s group confirmed that surgical manipulation of human vein segments results in a significant increase in monocyte adhesion.40 However, direct evidence linking improved endothelial integrity to a reduction in intimal hyperplasia, enhanced outward remodeling, or improved patency is lacking. Nonetheless, significant indirect evidence supports the concept that endothelial preservation minimizes the early biologic perturbations within vein grafts, and therefore harvest techniques aimed at reducing endothelial dysfunction seem reasonable.
Dissection
Precise, atraumatic dissection and minimal direct manipulation of the vein via a “no-touch” technique have been demonstrated to reduce endothelial damage during harvest (Fig. 92-2).41–44 Though somewhat a misnomer, the “no-touch” technique involves limited handling of the vein without direct application of forceps or vascular clamps. Major branches are ligated away from the wall to avoid narrowing of the lumen and promote outward remodeling after implantation. Opinions regarding the importance of maintaining continuity of the vein during dissection versus early ligation for intermittent infusion of isotonic fluids are mixed. Despite not being studied in a directed manner, continued continuity of vein has been shown to reduce the formation of small thrombi on the wall, and cannulation and division of the distal vein promote the direct application of antithrombotic and smooth muscle relaxants directly into the lumen. Although limited vein graft handling is universally considered an important component of promoting both short- and long-term graft patency, this concept is challenged by the nearly identical clinical outcomes for reversed, nonreversed, and in situ vein grafting techniques.45,46 If limiting vein manipulation is a dominant factor, one might intuitively expect improved outcomes with in situ grafting, where direct vein manipulation can be essentially eliminated, and compromised outcomes with nonreversed/excised grafts, which require complete mobilization with valvulotomy. The absence of such differences suggests that careful dissection of the vein is prudent, but absent extensive injury, it is of only modest importance to final outcomes.
Figure 92-2 “No-touch” technique used in the harvest of autogenous vein. To minimize spasm, papaverine (120 mg/L) is injected into the periadventitial plane before surgical exposure. Side branches are identified and ligated several millimeters away from the wall to prevent luminal narrowing after implantation and outward remodeling of the vein graft. Using a Silastic vessel loop to control the vein without direct manipulation with surgical instruments, the periadventitial tissues are divided and the vein is excised.
Though not examined in the setting of peripheral vein grafts, saphenous vein encased by a thick pedicle of surrounding adipose tissue has been used for coronary bypass grafting with promising results. Morphologic and physiologic studies have confirmed reduced endothelial injury and increased expression of endothelial nitric oxide synthase when compared with the standard technique for vein harvest.47,48 Prospective randomized clinical testing has demonstrated improved short- and long-term patency with use of the pedicle harvest technique, with 90% versus 76% patency rates at 8.5 years for pedicle versus standard grafts.49,50 Although this technique may not be applicable to the long-segment grafts required for lower extremity revascularization, it is interesting to speculate on the underlying mechanisms for these improved outcomes, which could include decreased endothelial injury, maintenance of an intact vasa vasorum, or the potential delivery of biologic mediators from the surrounding periadventitial tissue.
Distention Pressure
Cannulation of the vein is required before implantation to evaluate the potential diameter of the graft, assess for leaks, and identify adventitial bands compromising the lumen. Use of a standard small-volume syringe can produce intraluminal pressure in excess of 700 mm Hg.51 A number of investigations have examined the effect of distention pressure on endothelial integrity and almost uniformly have observed that pressure in excess of 100 mm Hg causes patchy endothelial denudation and that pressure in excess of 500 mm Hg leads to disruption of the underlying media.52–54 The intrinsic biomechanical properties of the graft are negatively impacted, with a resultant decrease in wall compliance secondary to disruption of the elastic elements within the wall.55 Biologic function is also influenced, with distention injury initiating an increase in c-fos expression, an upstream regulator of platelet-derived growth factor production.56 Among the few studies to contradict this observation is the work of LoGerfo et al,54 who observed no significant injury up to a distention pressure of 500 mm Hg and speculated that their aggressive use of papaverine prevented vasospasm and minimized the effect of overdistention. Nevertheless, a variety of pressure-sensing devices have been developed to allow the surgeon to monitor or control distention pressure. Ranging from syringes with intrinsic pressure transducers to reservoir inflation bulbs that generate a fixed pressure, these devices have undergone only sporadic testing and have never been accepted into widespread clinical use.
Irrigating Solution
An array of irrigating solutions ranging from crystalloids to colloids to autogenous blood has been used during vein harvest. Among the first and still the mostly widely used solutions are the isotonic crystalloids normal saline (pH 5.5, 308 mOsm), lactated Ringer’s (pH 6.5, 273 mOsm), and Plasma-Lyte (pH 7.4, 292 mOsm). Although the extent of injury and the magnitude of biochemical perturbations with these solutions are variable and depend on confounding variables such as temperature and the use of pharmacologic adjuncts, the bulk of the literature would suggest that simple crystalloid solutions are most damaging to the endothelium.39,42,51,57 Modified crystalloid solutions such as tissue culture and whole-organ preservation media have also been investigated and have demonstrated modest reductions in endothelial denudation and no improvement in endothelial function when compared with an isotonic saline perfusate.54,58,59 Without ready access to these solutions in the operating room and because of only modest benefit, these modified crystalloids have not been widely accepted for clinical use. Despite some concern for fibrin deposition, predominantly at higher temperatures, heparinized autologous blood probably provides the best option for use during vein harvest.39,42,51 Published reports, however, are not unanimous, with several suggesting equivalent results when using standard crystalloid solutions with pharmacologic adjuncts.58,60
Temperature
Focusing on maintaining endothelial integrity, LoGerfo et al put forward the concept that warmer perfusate temperatures during active dissection would prevent vasospasm, whereas storage of the graft and minimization of metabolic insult would be best accomplished at a much cooler temperature.54 Supporting this hypothesis, they demonstrated that infusion of 37° C perfusate during active vein harvest and storage of the distended vein at 4° C minimized the extent of endothelial damage. Notably, the influence of this dual-temperature approach was most prominent in saline-treated grafts. Subsequent investigations using a single-temperature approach also favored perfusion with a 4° C irrigation solution,39,54,57 with blood preferred over saline because of the mural edema associated with cold saline infusion.42
Although initial studies focused on endothelial morphology as the primary endpoint, further work in the field diversified to examine the effect of solution temperature on biologic activity of the vein graft wall. Nitric oxide–mediated vasodilatation is greatly impaired after storage at 4° C, with room-temperature blood and Plasma-Lyte demonstrating little deterioration when compared with control veins tested immediately after harvest.61 Analogous studies examining endothelial prostacyclin production and functional thrombomodulin activity support the use of room-temperature perfusates as the best option for preserving metabolic activity.62,63
With conflicting experimental evidence, delineation of the most favorable storage temperature is difficult, and reasonable arguments for either cold- or room-temperature treatments can be crafted. Although no definitive recommendation can be proposed, the increased effort required to supply and maintain cold perfusate in the operating suite has prompted most clinicians to favor the use of room-temperature solutions.
Pharmacologic Adjuncts
Unfractionated heparin has universally been included in most vein harvest solutions. Aimed at reducing fibrin deposition and the formation of microthrombi, doses ranging from 4 to 10 U/mL are typically used.39,57,64
Prevention of vasospasm is widely identified as being critical in maintaining an intact endothelium, and the use of pharmacologic agents to facilitate this goal may be the most important component of the vein harvest regimen. The most widely studied vasodilator for this purpose is papaverine. Although its exact mechanism of action is unclear, papaverine appears to induce smooth muscle cell relaxation through inhibition of phosphodiesterase and an increase in intracellular cyclic adenosine monophosphate levels. Percutaneous injection of papaverine (120 mg/L) along the outside of the vein before skin incision has been described as an important step to minimize spasm,64,65 although this can prove challenging in all but the thinnest patients. Application of papaverine along periadventitial tissues and within the lumen perfusate is more easily accomplished and continued throughout vein harvest. Though not rigorously studied as an independent variable, most regimens containing papaverine have shown reduced endothelial injury in comparison to controls.39,54,57,60
Other vasodilators have been examined, and a combination of glyceryl trinitrate (8.3 mg/L) and verapamil (16.7 mg/L) appears to be particularly beneficial.66 In direct comparison to papaverine, glyceryl trinitrate/verapamil demonstrated notable improvement in endothelial coverage.
Even though extensive research has been conducted in this area, no consensus has been reached regarding the optimal techniques for vein graft harvest, with each variable demonstrating unique advantages and shortcomings. Box 92-1 provides a practical summary protocol for the preparation of autogenous vein conduit. The relative importance of these variables is best illustrated by the work of Adcock et al, who examined the combined benefits of papaverine, autologous blood, and limited pressurization in maintaining endothelial integrity.39 Even though this combined regimen achieved a significant reduction in endothelial loss at the time of implantation, 71% of the endothelial cells exhibited morphologic abnormalities by the second postoperative day (versus 92% for the “standard” regimen). Although the majority of published regimens have sought to optimize variables to maintain an intact endothelial monolayer at the time of implantation, few reports have challenged their techniques to extended exposure in an in vivo environment. Widespread endothelial injury and metabolic perturbation of the harvested vein are probably unavoidable, with only secondary improvements gained by the various methodologies just described.
Minimally Invasive Vein Harvest
Among the significant complications associated with a single continuous incision for lower extremity vein harvest are wound infection and dehiscence, observed in up to 40% of patients.67 Minimally invasive approaches to saphenous vein harvest have been developed to reduce incisional length and diminish the attendant morbidity. Early investigations of this technique were initiated without specialized instrumentation but used a series of small, sequential incisions overlying the vein. The use of such “skip incisions” significantly improved primary wound healing, with wound complications developing in 28% of patients with a continuous incision as opposed to 9.6% of patients in the “skip incision” group.68 Over the past 2 decades the concept has evolved with the marriage of endoscopic visualization and specialized instrumentation to facilitate minimally invasive vein harvest. Several different devices have been developed for this purpose, all using three small incisions to access the vein and ligate side branches. Used predominantly for harvesting of vein for coronary bypass surgery, prospective randomized human trials have demonstrated significant reductions in wound morbidity when compared with single-incision saphenectomy.69–71 Increased manipulation of the vein is inherent with this approach and raises concern about increased endothelial damage and accelerated graft failure. Blinded morphologic examination of harvested human vein specimens72,73 and clinical outcome studies71 have demonstrated no significant differences in vein injury or graft patency. Universally noted throughout these reports is the steep learning curve that accompanies endoscopic saphenous vein harvest, with many centers identifying a core group of practitioners who focus on this aspect of the procedure. Although performance of these studies at large-volume medical centers can provide insight into the optimum outcomes with this approach, extrapolation to lower volume centers may not be as promising.
Probably related to the long segments of vein required for lower extremity bypass and the prolonged learning curve inherent in this approach, endoscopic vein harvest for peripheral revascularization has not been uniformly embraced. A small number of centers have championed its use, with mixed results. With no randomized trials comparing single-incision with endoscopic saphenous vein harvest for peripheral bypass, experiences have predominantly been reported through case series with retrospective controls.74–78 These reports generally support the observations in the cardiac literature and suggest that endoscopic harvest offers reduced wound complications with no notable deterioration in short- and long-term graft patency. Not all reports have been favorable, however, with one investigative team detailing inferior patency rates with little improvement in wound complications after endoscopic vein harvest.78 Among the important conclusions in this study is the significant learning curve inherent in this technique, with an estimated 40- to 50-patient experience required to become fully competent in harvesting the more difficult below-knee saphenous vein.79,80 It is noteworthy that the center reporting inferior results performed an average of only one endoscopic harvest per month, probably an insufficient volume to master this technique. Concerns about increased cost of the procedure secondary to additional equipment expenses are relatively unfounded, with these costs more than offset by decreased length of stay during the primary admission and a reduction in the number of readmissions for wound complications.76
In an effort to reduce instrument costs while retaining the benefits of a minimally invasive approach, a limited-incision saphenectomy was proposed in which 2-cm-long incisions flanked by 6-cm skin bridges were used to extract the vein with standard surgical instrumentation. When compared with historical controls, this limited-incision approach offered no improvement in wound complications and a notable reduction in long-term patency.78 The authors speculated that the significant manipulation of the vein required for exposure beneath the skin bridges is the probable cause of the inferior performance of these grafts.
Autogenous Vein Graft Configurations
In the preoperative planning stage before open lower extremity revascularization, the surgeon is faced with a number of variables concerning the suitability of various inflow and outflow arteries and the length and quality of the available conduit. Invariably, because of some discordance among the available options, assessment of the potential risks and benefits for each choice is required. Preoperative arteriography and duplex vein mapping provide the baseline data through which primary and secondary operative plans are developed. Direct operative assessment of the intended proximal and distal anastomotic sites and the quality of the autogenous conduit may initiate reevaluation and modification of the intended plan during the course of the operation. A summary of the various factors that influence this decision is provided in Table 92-1.
The conduit of choice is the ipsilateral great saphenous vein, with the contralateral saphenous vein being an appropriate secondary option. To preserve the great saphenous vein in the contralateral limb for future revascularization procedures, some surgeons have advocated the use of alternative conduit as a secondary option.81 Studies examining this issue are in general agreement that in the absence of advanced ischemia (disabling claudication, rest pain, or tissue loss), use of the contralateral saphenous vein is recommended over alternative conduit because of its length, superior performance, and minimal risk to the donor limb.82–84 Independent risk factors predictive of the need for future vascular intervention in the contralateral limb include age younger than 70 years, diabetes, coronary artery disease, and an ankle-brachial index (ABI) of less than 0.7. In patients demonstrating three out of these four risk factors, the potential for requiring contralateral revascularization within the next 5 years ranges from 25% to 43%, and use of alternative conduit over the contralateral saphenous vein may be a reasonable consideration in this select population.85
The approach of using the “best” available autogenous conduit has been advocated86; this practice is used routinely by most vascular surgeons. Although vein segments with chronic phlebitis, calcification, or organized intraluminal thrombus are prone to accelerated failure and should be excluded from use,16,17 the other characteristics that define the “best” vein have been an active area of investigation. Even though single-segment saphenous vein has universally been identified as the most durable conduit, an array of single-institution, retrospective studies have attempted to define the effect of such characteristics as vein diameter, graft type, and orientation on long-term patency.11,31,87–91 Though useful in framing this question, the most comprehensive examination of this issue can be obtained from the prospective, multi-institutional PREVENT III (Project of Ex-vivo Vein Graft Engineering via Transfection) database.32,92 Examining conduit diameter and using veins greater than 3.5 mm as the reference standard, this trial found that grafts ranging from 3.0 to 3.5 mm had a 1.5-fold risk for primary failure. Grafts smaller than 3.0 mm in diameter had a 2.4-fold higher risk for primary failure and a 37% loss of secondary patency at 1 year. In terms of graft type, composite veins had a 1.5-fold increase and arm veins a 1.6-fold increase in primary failure when compared with single-segment great saphenous vein. Graft orientation did not influence patency, with reversed and nonreversed configurations demonstrating equivalent patency rates. As these data demonstrate, deviation from using an adequately sized single-segment saphenous vein carries increased risk for failure, and understanding the magnitude of these risks is critical in operative planning and maximizing the potential for a durable outcome.
Reversed Vein Grafts
Excision of the vein with orientation in a reversed configuration while maintaining antegrade flow through intact valves offers the most straightforward method for vein graft implantation and is suitable for most clinical situations. Difficulty may arise when significant tapering of the saphenous vein in the distal part of the limb creates a size mismatch between the artery and vein graft at both the proximal and distal anastomoses. The proximal vein graft appears to be a common site for luminal narrowing and vein graft failure, and use of small-diameter conduit at this location may accentuate this problem.93 The size mismatch can be most problematic, however, when performing a tibial or pedal artery anastomosis. A fourfold to fivefold difference in diameters may be encountered, and tailoring the proximal great saphenous vein into a suitable anastomotic configuration can be challenging in these situations.
Examination of normal venous physiology has demonstrated that complete effacement of the valves does not occur during periods of prograde flow. In the low-flow, nonpulsatile hemodynamic environment that characterizes the superficial venous system, the mild to moderate stenosis created by these partially open valves does not translate into a significant loss of pressure or impediment to flow. After implantation into the arterial system, the hemodynamic significance of the valves becomes more pronounced. Though not significantly narrowing the lumen during peak systole, a transition to turbulence with localized regions of stasis is noted within the valves.94 Lysis of these valves leads to a 15% decrease in hydrodynamic resistance and a 15% to 30% increase in graft flow rates.95–97 Intact valves have not been proved to adversely influence the long-term patency of reversed vein grafts, but based on these observations, routine valve lysis within these antegrade flow grafts has been proposed.
Less well studied is the biologic effect of the hemodynamic microenvironment within the valve regions, which serve as a nidus for the development of a hyperplastic lesion and focal graft narrowing. Strandness et al prospectively evaluated the long-term remodeling of these valves in reversed saphenous vein grafts.98,99 Ten percent of the valves were associated with greater than 50% stenosis, but only 2.5% progressed to a critical stenosis requiring operative intervention; these valves accounted for 17% of the total graft revisions within the 18-month follow-up period. Notably, valve morphology was dynamic, with 60% of the hemodynamically significant valve lesions showing regression to less than 20% stenosis in a mean period of 3 months.
Nonreversed Vein Grafts
Challenges in constructing a reversed-configuration vein graft include significant proximal-to-distal tapering of a vein and the resulting size mismatch at the proximal or distal anastomoses (or both). Although use of an in situ grafting technique can be one solution to this issue, the combination of complete excision of the vein, lysis of the valves, and orientation in a nonreversed configuration offers an alternative technique. Despite the fact that initial attempts with this approach suggested results inferior to the in situ method, these early efforts were probably compromised by the use of an eversion valvulectomy technique and extensive injury to the vein wall.100 With subsequent refinements in the approach to valve lysis, nonreversed vein grafts have proved as durable as other available techniques.101–104
Although nonreversed vein grafts inherently require increased manipulation with the potential for injury during valve lysis, this shortcoming is theoretically offset by the improved hemodynamics offered by this approach. After valve lysis, nonreversed grafts offer a 20% improvement in flow rates over nonlysed, reversed conduits.96 This hemodynamic effect is most pronounced in smaller diameter vein grafts ranging from 2.0 to 2.5 mm, where the leaflets from intact valves encompass 45% of the luminal cross-sectional area.95
In Situ Vein Grafts
The concept of using the great saphenous vein as a graft with mobilization of only the proximal and distal segments while maintaining the interval region within its subcutaneous bed was initially suggested by Rob’s group in 1959.105 Interested in the potential hemodynamic advantages offered by removal of the valves and retrograde perfusion of the conduit, Rob believed the procedure to be too time consuming for routine application. Hall, a visiting fellow from Norway, became intrigued by this approach, refined the techniques for routine clinical application, and published the first report of the in situ vein graft technique in 1962.106 His version of the procedure, requiring valve excision by opening the vein at multiple locations along the graft, was tedious, required significant technical expertise, and failed to gain widespread acceptance. The development of efficient instrumentation for valve lysis provided the opportunity to reduce vein manipulation and maintain an intact vasa vasorum and fueled renewed enthusiasm for the in situ approach. Initial reports of this approach, championed by Leather et al,107 suggested improved patency over excised vein grafts.108 These early analyses are compromised by the use of historical controls, however, and contemporary comparisons of in situ versus reversed or nonreversed grafts fail to demonstrate significant differences in long-term outcomes.46,103,109
Preparation of the saphenous vein for use in situ as an arterial bypass entails (1) mobilization of the proximal and distal segments for construction of the anastomoses, (2) removal of the valvular obstructions to arterial flow, and (3) interruption of the venous side branches to prevent the formation of arteriovenous fistulae. The objective is to accomplish these tasks in the most expeditious manner while minimizing traumatic injury to the vein.
Proximal and Distal Anastomoses
The common femoral artery serves as the most common source of inflow for infrainguinal revascularization, and the relative position of this artery and the confluence of the superficial inguinal veins dictates construction of the proximal anastomosis. Mobilization of the proximal great saphenous vein is accomplished by secure ligation of the superficial branches, placement of a side-biting vascular clamp across the common femoral vein, transection of the great saphenous vein flush with the wall, and repair of the femoral vein with running nonabsorbable suture. Lysis of the most proximal great saphenous valve can be difficult with standard valvulotomes, but localized eversion of this segment permits excision of the valve under direct vision. Intrinsic atherosclerotic disease in the common femoral artery may dictate more proximal placement of the anastomosis. Additional proximal vein length can be obtained by harvesting a segment of the superficial epigastric vein and extending the venotomy proximally along the posterior surface of this vein to provide an autologous patch for repair of proximal common femoral artery disease. Care should be taken to minimize longitudinal stretching of the graft, which can accelerate the development of intimal hyperplasia.110 Extensive common femoral or profunda femoris origin disease (or both) may necessitate endarterectomy with patch angioplasty or graft replacement of the common femoral artery. In the absence of significant proximal artery occlusive disease, defined as greater than a 50% reduction in lumen diameter, the superficial femoral, profunda femoris, or popliteal artery can be considered for placement of the proximal anastomosis.111
The posterior tibial, peroneal, and dorsalis pedis arteries provide the most straightforward options for placement of the distal anastomosis. Although the below-knee popliteal artery can also be used, fashioning the anastomosis can be problematic because of the nearly 90-degree angle that is formed between the graft and artery. With most hemodynamic analyses detailing an increase in flow separation and activation of proliferative metabolic pathways with right-angle anastomoses, grafts configured to this segment of the popliteal artery may benefit from excision and placement in an anatomic tunnel. Distal anastomoses to the above-knee popliteal artery do not offer the same constraints on their configuration; however, after mobilization of the proximal and distal graft, the segment that remains in situ can be quite short and of limited benefit.
Valve Lysis
Although limited transverse venotomies were used in initial development of the in situ bypass graft, this approach to render the valves incompetent has given way to less invasive techniques. Valvulotomes have undergone a variety of revisions over the last several decades, and the current generation of devices can be classified into three categories—the modified Mills valvulotome, the adjustable valvulotome, and the fixed valvulotome (Fig. 92-3).
Figure 92-3 Valvulotomes. A, Modified Mills. B, LeMaitre adjustable. C, Uresil fixed with 2-, 3-, and 4-mm cutting heads.
The modified Mills valvulotome (see Fig. 92-3A) was initially described in 1976 for application to coronary artery bypass surgery.112 With limited modification, this device was rapidly adopted for use in peripheral vascular surgery. Introduced into a side branch or the distal end of the vein, the device is advanced through the valve leaflets (Fig. 92-4). The proximal vein graft is distended by arterial inflow or manual infusion to induce valve closure, and the valvulotome is slowly withdrawn until the reverse cutting blade engages the valve leaflet. The tip of the valvulotome is maneuvered toward the center of the lumen, and a short burst of inferior traction is applied to transect the valve.
Figure 92-4 Valve lysis using the modified Mills valvulotome. The valvulotome is inserted into a long side branch and advanced into the proximal vein. Competent valves within the graft are maintained in closed position by arterial inflow pressure, and the valvulotome is withdrawn until resistance is encountered. After ensuring that the instrument has not engaged a side branch, a short burst of inferior traction is delivered and the valve leaflet transected. Short advancement and 180-degree rotation of the valvulotome permit division of the opposite valve in analogous fashion.
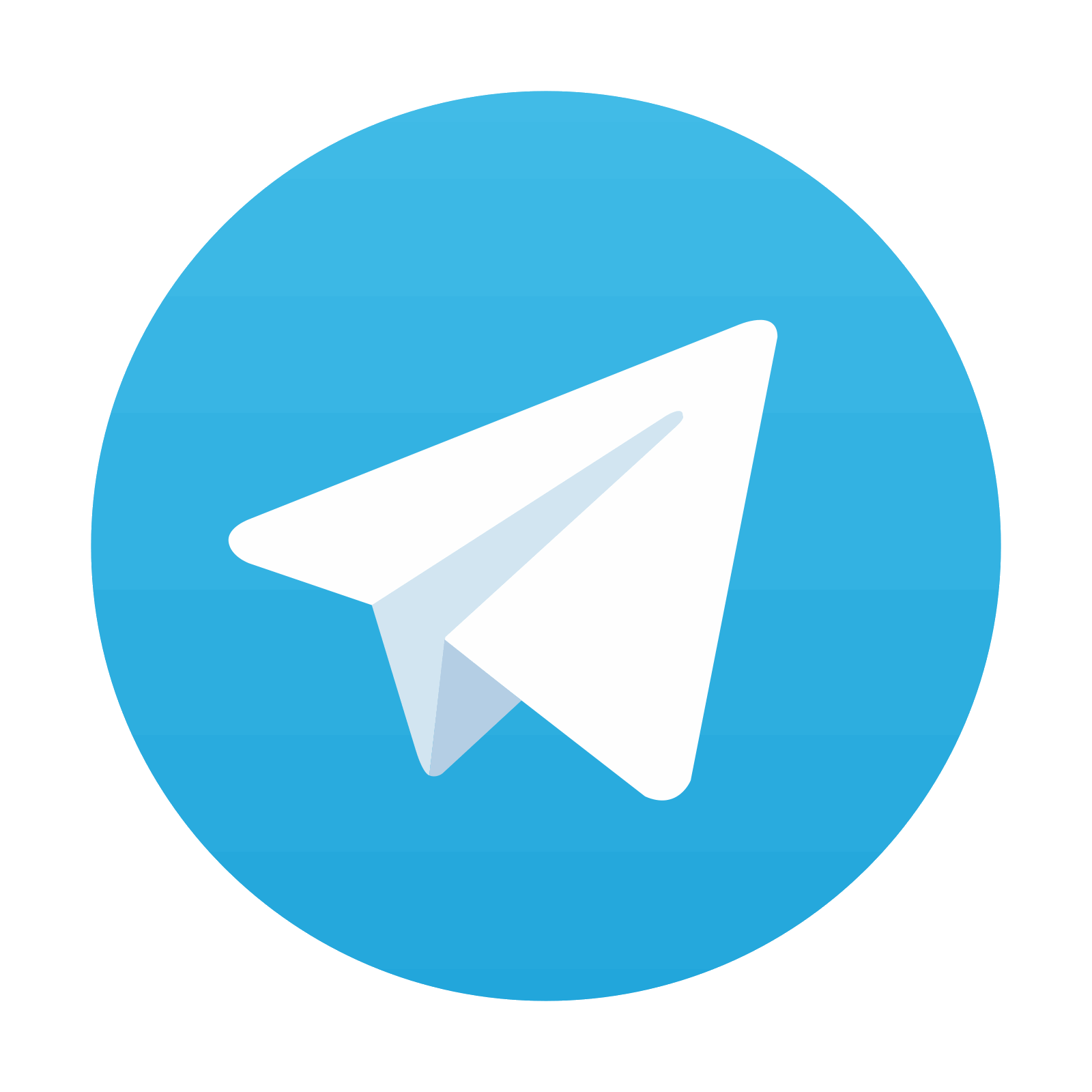
Stay updated, free articles. Join our Telegram channel
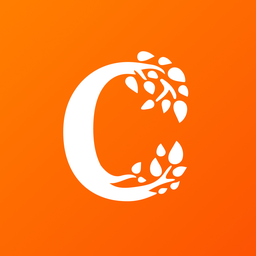
Full access? Get Clinical Tree
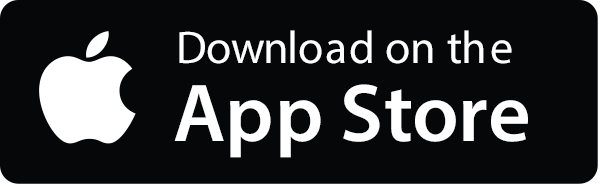
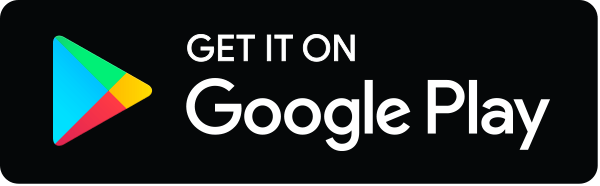