Keywords
Vestigial circulationMean circulatory pressureBlood’s “motor energy”Cardiac arrestSpontaneous return of circulationRete mirabileNegative interstitial pressureInterstitial spaceTotal body waterWound VAC therapyNegative pressure pulmonary edemaNegative intrapleural pressureMechanical lung ventilationThe mean circulatory filling pressure (MCP or MCFP) is a dynamic measurement which can be extrapolated from the rate of rise in venous pressure when the activity of the heart is suddenly stopped, and the pressure is equilibrated from the aorta to the right atrium. As mentioned, an accurate measurement of MCP is difficult to obtain in laboratory animals because of numerous compensatory mechanisms that are elicited at the time of induced cardiac arrest (for review, see [1]). The question naturally arises to what extent is such a radical intervention representative of the actual events at the level of the microcirculation, accounting for the fact that the distribution of flow is tightly bound to metabolic demands of the tissues and/or individual organs? The only substitute for such “flow averaging” is to examine the actual phenomenon itself, i.e., the capillary flow at this critical juncture in the life of the experimental animal or the human subject. New methods for direct observation of the microvascular beds during normal conditions as well as in critical illness have provided valuable new insights into microvascular dynamics (cf. Chap. 21).
However, such information will not be able to give us an answer about the actual conditions, unless we are acquainted with the nature of the underlying phenomenon. We will now examine two separate groups of “near death” circulatory phenomena: the first pertaining to venous and the latter to arterial circulations. Please note that in this context, the term “venous circulation” encompasses the entire compartment occupied by the venous blood including the pulmonary arterial system. By analogy, the “arterial” compartment comprises the rest of the circuit containing the arterial blood.
15.1 Phenomenon of Vestigial Circulation
Early attempts to acquire measurements of “static pressure” were carried out on “recently dead dogs” [2–4], and “recently deceased” patients, by a method of trans-thoracic cardiac puncture, as described by Starr [5]. Nevertheless, it is evident, from Starr’s paper that more was afoot than expected, when he comments that “Occasionally small rhythmic fluctuations in the static blood pressure made one wonder if all vital activity had ceased. Similar fluctuations were seen in the animal experiments. In a number of instances, I attempted to demonstrate a persistence of vascular activity in the patients by injecting histamine and adrenalin into the skin. There was no visible response in any case” [5]. Similarly, Guyton in one of his early papers submits that “The mean circulatory filling pressure as it exists in the body at any given instant cannot possibly be measured because life is dependent on dynamic circulatory conditions” [2]. Despite the obvious ingenuity of Guyton’s technique for repeated measurements of MCP in dogs by which a rapid equilibration of volumes (and pressures) is possible, there remains a problem. The experiment was evidently designed to support the concept of MCP in the venous return model and, as such, did not consider more subtle phenomenology that accompanied it.1
In the 1940s, Thompson investigated the possible beneficial effect of negative pressure during the expiratory phase of mechanical ventilation in a dog model. Anesthetized, intubated, and heparinized dogs were asphyxiated by clamping of the endotracheal tube, resulting in demise by asphyxia in 8–10 min. Thirty minutes after the cardiac arrest, mechanical ventilation and oxygenation were resumed and continued for 1 h. To assess the residual movement of the blood, if any, tracer substances (radioactive sodium and fluorescein) were injected and sampled at predetermined intervals, and hemoglobin oxygen saturation was measured. The results showed a gradual rise in arterial oxygen saturation up to normal levels, signifying a continuous movement of blood through the pulmonary and systemic circulations. Concentrations of dye and radioactivity were measured in various organs and reported—confirming their distribution according to perfusion—but no quantitative data on blood flow was given. Non-heparinized, non-ventilated controls were also run, in which no significant movement of substances was detected, possibly due to blood clotting. Thompson concluded that mechanical ventilation as such (without the action of the heart) is the cause of significant residual circulation, which is enhanced by introducing of a negative expiratory phase during mechanical ventilation [6].
These experiments were repeated in the 1960s by Manteuffel-Szoege and coworkers on three groups of dogs. Oxygen saturation in arterial and venous bloods was measured as an indicator of blood’s movement. In the first group, where mechanical ventilation was resumed 30 min after hypoxic cardiac arrest, the arterial O2 saturation levels reached 100%. An estimation of blood circulation time gave the values in the range of 5–8 min (dye dilution technique), which is some 20–30 times longer than in normal controls. The estimated circulating blood volume in 6 of 13 dogs showed values of 105–962 mL. In the second group, oxygen was insufflated (without mechanical ventilation) via the endotracheal tube 10–15 min after cessation of the heartbeat. The arterial oxygen saturations increased from a baseline of 20–30% to 85%. In the third group, neither ventilation nor insufflation of oxygen was given. In these dogs the dye injected intravenously was not recovered in the arteries; however, observation of the omental vessels confirmed blood movement lasting up to 2 h [7, 8].
Manteuffel-Szoege performed further experiments on anesthetized and curarized dogs with an open chest in which both venae cave and aorta were cross-clamped simultaneously for over 2 h. A “pressure reversal ” was observed in which the brachial artery pressure dropped to zero and the caval pressure rose to 13 mmHg. While the blood continued to flow in the normal direction, it flowed against the pressure gradient. The peculiar phenomenon of “pressure reversals” was also observed by Manteuffel-Szoege on patients during open heart surgery in moderate and deep hypothermia and also in dogs [9, 10]. Several other experiments were performed on dogs in deep hypothermic circulatory arrest (core temperature 10 °C). After the extracorporeal circulation was stopped and both venae cavae and the aorta were occluded, the blood continued to flow in the small arteries and capillaries of the omentum for 15–30 min, whereas flow in the veins soon came to a halt. No vasomotor activity was observed due to deep hypothermia. The phenomenon of pressure reversal has also been demonstrated, but not recognized as such in the pulmonary circulation [11].
Manteuffel-Szoege maintained that the phenomena of pressure reversal (in larger vessels) and continuation of flow in the normal direction (but against the pressure gradient) at the level of the microcirculation suggest that the blood has its “own motor energy ,” which persists for some time in spite of increased viscosity and “sludging” of blood at low temperatures. He proposed that this motive energy is “inseparably connected with thermal conditions of the animal” [12, 13]. The phenomenon of vestigial circulation was further demonstrated by excising a portion of the omentum of a dog in which “intensive blood movement could be observed for 2–3 min and slower movement up to 10 min after the excision.” Rapid removal of parenchymal organs such as hearts and kidneys from dogs demonstrated bleeding (up to several minutes) from the veins and “gaping empty arteries.” In an excised liver the blood flowed from hepatic veins while the portal vein was empty [7]. Based on those and additional experiments on the chick embryo (mentioned in Chap. 8), Manteuffel-Szoege suggested that the movement of blood against the pressure gradient lends further support to the fact that the blood possesses its “own kinetic energy.” Evidence for the vestigial movement of venous blood is further supported by the demonstration of congestion of the great veins and distension of the heart after circulatory arrest in asphyxiated, open-chest dog, and seen clinically as distension of neck veins in patients dying of cardiac arrest [9].
A recent animal study entirely confirmed the residual blood movement following the cessation of effective myocardial contractions. Skulec and coworkers observed sublingual microcirculation in two groups of pigs after induced ventricular fibrillation (VF) or pulseless electrical activity (PEA) by means of side stream dark-field imaging (SDF) in vivo microscopy. The animals were left in a non-resuscitated state until the cessation of cardiac activity monitored by transthoracic echocardiography. The results showed persistence of blood’s movement in the capillaries in PEA animals up to 31 min (23.2 ± 8.7) and up to 40 min (25.3 ± 12.6) in the VF group. The authors concluded that the observations support the concept of autonomous streaming of the blood at the level of the microcirculation subject to local metabolic control which continues a significant amount of time after the “collapse” of macro-circulation [14]. Resuscitation protocols have been developed which specifically enhance the microcirculatory flow, such as the use of sodium nitroprusside—an NO− donor [15].
Additional evidence for vestigial movement of blood after clinical death comes from forensic pathology where uneven distribution of blood within individual organs has been documented. For instance, the distribution of blood in the kidney of victims dying suddenly from electric shock shows a tendency for the blood to collect in the venous system. Similarly, in the victims of acute hemorrhage, the renal arteries and capillaries are empty, while renal cortical veins and venae rectae (of the medulla) are filled with blood [16].
It is significant in this context that the liver, receiving blood from the abdominal organs, is on the downstream of the systemic capillaries supplying these organs with arterial blood. The liver capillaries, as the final ramifications of the portal vein, thus represent a second set of capillaries, the so-called rete mirabile. They are placed in series with capillaries of the abdominal organs. Considering that the liver veins drain into the inferior cava with pressures close to those in the right atrium, it is surprising that within the pressure propulsion paradigm, the heart can overcome such an amount of added resistance. The situation is similar in case of the kidney where the peritubular capillary network lies downstream to the glomerular capillary plexus.
The fact that arteries are free of blood after death, unlike veins, was already known by the ancient Greeks. In an 1850s review on the causes of emptiness of the arteries after death, London pathologist Thudichum noted that, “I, for my own part, never met with any blood in any artery in several hundred dissections, in the 5 years during which my attention has been directed to the subject” [17]. Even though the importance of this seemingly trivial observation seems to have fallen by the wayside in more recent times, it continues to make its appearance under different guises and has been difficult to ignore. For example, it is generally known by the forensic toxicologists that significant redistribution of substances (drugs) occurs postmortem. Ample evidence exists that concentration of drugs in the central circulation can be several times greater than in the peripheral circulation [18]. Animal models show that significant distribution of drugs occurs up to 24 h postmortem [19] Although the uneven distribution is ascribed to diffusion, this would clearly have to occur against the concentration gradients. Thus the unrecognized existence of vestigial circulation presents an ongoing dilemma in human toxicology, expressed by one of the authors as, “We conclude that this poorly studied phenomenon creates major difficulties in interpretation and undermines the reference values in the data bases where the site of origin of post-mortem blood samples is unknown” [20].
The phenomenon of spontaneous return of circulation (SROC) after failed resuscitation is a medical curiosity that has likewise eluded a rational physiological explanation. It was first described in the literature in 1982 and is popularly known as “Lazarus syndrome” or auto-resuscitation. To qualify, the patients must have experienced a monitored asystole or pulseless electrical activity, from which they could not be resuscitated, followed by an unexpected, spontaneous return of circulation. In a recent review of 38 cases, collected mainly from the anesthesia and intensive care journals, 14 patients had complete neurological recovery, in five patients it was considered “good” and the rest died of complications [21]. Various explanations for the phenomenon have been proposed such as, among others, artificial ventilation, “auto-PEEP,” due to dynamic hyperinflation of the lung during CPR and delayed drug action, none of which are generally accepted [22]. The authors stress that death is not an event but a process during which various organs supporting the continuation of life fail. They further maintain that the absence of pulse and respiration alone are not a sign of definitive death [21]. There continues to be a lack of consensus on the time interval between the termination of CPR and diagnosis of death [23]; however, recent studies employing the point-of-care echocardiography have confirmed that contractile activity of the heart is frequently present in patients with no peripheral pulses, a condition known as pseudo-PEA (pulseless electrical activity) [24] and even in patients with asystole [25]. The introduction of portable echocardiographic devices not only allows for direct visualization of the “arrested heart” but enables to follow its response to mechanical and chemical interventions during CPR. The currently accepted algorithm of “high quality CPR” with adequate depth, and the rapid rate of chest compressions with minimized interruptions, clearly aims at sustaining of adequate flow/pressure to the heart and the brain [26]. In the context of the proposed circulation model, this protocol also prevents over-distension of the thin-walled right ventricle, known to occur not only in cases of pulmonary embolism, but also in cases of hypoxia and ventricular fibrillation [27].
We have seen that the attempts to measure MCFP under “steady conditions” by stopping the heart and rapid equilibration of blood among the vessels obscures the phenomenon of vestigial circulation which can persist for several hours after the cessation of the heartbeat. The concept of MCFP is therefore more than just a hypothesis which, according to the proponents of the left ventricular centered view of the circulation is inconsistent with their model of circulation but plays a dynamic role in the intact circulation, as argued by proponents of the venous return (VR) model of circulation. As proposed by Manteuffel-Szoege, the phenomenon is a residuum of blood’s own kinetic energy and confirms its autonomous movement. It is moreover apparent that existing models of circulation fail to give a consistent answer to questions about the relative role of the heart and of the peripheral circulation in the regulation of cardiac output.
15.2 Negative Interstitial Pressure

Schematic illustration of the interstitial space. The interstitium is organ and tissue specific and contains various proportions of blood vessels and lymphatic channels embedded in a matrix of hyaluronan and proteoglycans, supported by collagen and elastic fibers. Cellular elements include a mix of fibroblasts, mast cells, macrophages, neutrophils, and plasma cells. Negative interstitial pressure assures the patency of microvascular beds and maintains normal tissue turgor

Distribution of total body water (TBW). Approximately 60% of body weight (42 L in a 70 kg adult male) consists of water (the amount is about 50% in females due to higher content of adipose tissue). Sixty percent or 25 L of TBW is distributed within the cells (intracellular fluid) and the rest (17 L) in the extracellular compartment, which consists of blood (5 L) and interstitial fluid (12 L)
The majority of IS water is “bound” to the above-mentioned gel-like ground substance, but a small amount is believed to be “free.” The hydrostatic pressure of this free water is defined as interstitial fluid pressure (IP) [30]. Studies employing deuterium oxide as a marker have shown that the exchange of water and solutes across the capillary membranes is in order of 80,000! L/day [31]. The IS is therefore a highly dynamic space whose composition profoundly affects the ionic gradients and function of the adjacent organs. The discovery in 1992 of specific water transcellular channels, the aquaporins, opened a new field of research in animal and human physiology [32]. To date, 13 transmembrane proteins have been discovered in virtually every organ system of the body [33]. In addition to water, the aquaporins facilitate transport of small solutes, including glycerol, urea, and carbon dioxide [34]. Experimental data shows up to 50-fold facilitation of aquaporin water transport across the cellular membrane [33]. Significantly, such large bidirectional fluid shifts can occur within minutes or seconds and far surpass those predicted by low-capacity, non-selective osmotic gradients [35].2

Lymphatic vessels. Terminal lymphatic vessel is a closed, capillary-like channel with open endothelial gap junctions and fenestrations, contiguous with the liquid phase of the interstitial space. Any increase in interstitial pressure facilitates net fluid flow into lymphatic channels in which the centripetal flow (red arrows) is maintained by one-way valves and rhythmic contraction of larger vessels

Estimation of the interstitial fluid pressure by Guyton’s method. A perforated Teflon ring is implanted subcutaneously, and the wound allowed to heal. The pressure in the capsule is measured by a hypodermic needle connected to a manometer (view from above, left). Side view of the implanted Teflon ring (right)
With the exception of some parenchymal organs, such as liver and kidney, negative IP exists to a varying degree throughout the body [28, 30]. In fact, it is because of the negative intrapleural pressure which keeps the lungs expanded, that the respiration is at all possible.3 Negative IP in the lung is of particular importance in facilitating resorption of edema fluid across the alveolar epithelium into the IS where it is rapidly cleared by the lung lymphatics [41]. The rapid and remarkable effectiveness of alveolar clearance can be witnessed in cases of acute pulmonary edema following upper airway obstruction, known as the negative pressure pulmonary edema. The event typically occurs during emergence from general anesthesia when the patient inspires against the closed glottis [42]. Sudden “flooding” of the alveoli with edema fluid can present with frothy sputum and a transient drop in oxygen saturation. The condition is self-limiting, responds to oxygen therapy, and usually clears within several hours. Excessive positive pressure applied to the lung, on the other hand, can be equally detrimental. Alveolar rupture from excessive positive pressure can result in tracking of air along the interstitial spaces into the mediastinum [43] or into subcutaneous tissue spaces [44]. A number of unusual cases of pneumomediastinum after dental extraction have been reported in the literature [45]. Here too, the entrainment and tracking of air via interstitial and perivascular spaces may be caused by the negative IP.4
Certain organs such as the brain and the kidney, enclosed with tight capsules, have positive parenchymal pressures. However, with respect to the atmospheric pressure, their interstitial pressures are negative [37]. Thus, not only the cerebrospinal fluid surrounding the brain but also the pressure in venous sinuses is sub-atmospheric (cf. Sect. 24.3).
The situation is similar in the eye which has (normal) positive intraocular pressure (12–14 mmHg), whereas the interstitial pressure within the cornea is negative. Experimental keratotomy and subsequent corneal flap reattachment generate suction forces in the range of −60 mmHg [46]. This is the force holding the cornea in place (without suturing) in patients after laser in situ keratomileusis (LASIK) surgery.
The negative IP presents the sum of forces between the lymph flow and capillary filtration/absorption and determines normal tissue and skin turgor. However, in case of increased negative pressure, the IS will “imbibe” (suck-in) water and contribute to edema formation. Experiments on animals show that a visible edema requires a doubling of interstitial fluid volume [47]. An active role of the IS has been demonstrated in (anesthetized) rat thermal injury model, where negative IP of −20 mmHg (burn of 10% body surface area) and −30 mmHg (burns of 40% BSA) have been reported [48]. Surprisingly, suction forces persisted in animals after the chemically induced cardiac arrest (intracardiac injection of KCl). When a plastic barrier was placed under the skin to prevent fluid transfer from the underlying skeletal muscle, negative IS pressures in the order of −95 to −135 mmHg (mean) were measured, with a maximum of −175 mmHg! [49]. Significant negative IP is moreover associated with certain forms of neurogenic inflammation, e.g., after electrical stimulation of the vagus [50], and in anaphylactic reactions [48]. Release of histamine and serotonin in a dextran-induced mast cell degranulation in a rat model of anaphylaxis resulted in a transient decrease in IS pressure (from −1.5 to −3 mmHg), soon to become positive (+1 mmHg) due to extravasation of plasma from the microcirculation. However, after induction of cardiac arrest, the IS pressure dropped further (to −10 mmHg) and persisted for up to 90 min. The authors proposed that the negative pressure was maintained due to the lack of blood flow as the source of additional plasma fluid [48].
Finally, the importance of negative tissue pressure has been recognized as a key aspect in wound healing. Originally described by Fleischmann [51] and gaining rapid acceptance after developing commercial application [52], the vacuum-assisted wound closure (VAC) has become a method of choice in the treatment of infected surgical wounds and of diabetic, decubitus, and venous stasis ulcers [53]. Although the physiological basis and effects of VAC is not fully understood [54], the restoration of negative tissue pressure attests to its effectiveness and underscores its importance in normal physiology. Some of the original authors have lauded the concept of VAC a “premature discovery” [55]; however, in light of the fact that the importance of negative tissue pressure, or vis-á-fronte, was recognized already by Greco-Roman physicians [56, 57], the VAC therapy should be considered a re-discovery. The question arises as to the methodology used by the physicians of the past that prompted them to introduce this concept, and more importantly, how to integrate it within the framework of contemporary knowledge (cf. Chap. 25).
In a comprehensive review article on the control of extracellular fluid volume, Aukland and Reed submit that “it is tempting to assume that regulation of interstitial fluid volume must be governed by some kind of monitor that could regulate capillary filtration/absorption through nervous control of capillary pressure” but conclude that such receptors have not been identified. Considering that this highly differentiated “peripheral” regulatory system with field-like quality would have to provide local control to various organs throughout the body, the existence of such a system, according to Aukland and Reed, is unlikely [28]. The situation differs in the case of the arterial pressure regulation, where the high-pressure baroreceptors (carotid sinus and aortic arch) and low-pressure baroreceptors (in the atria and the right ventricle) clearly provide and integrate the error signal in the brain stem and hypothalamus, respectively (cf. Chap. 24). Is it possible that Guyton’s venous return model of circulation, where the emphasis is placed on the relative independence of arterial and venous circulations (cf. Chap. 14), and the concept of negative IP [38] have a common link? It is proposed that the phenomenon of negative interstitial pressure is the evidence for the existence of such a global, but nevertheless locally active regulatory system. We will return to this subject in the final section of the book.
15.3 The Significance of Artificial Respiration: A Historical Overview
The method of artificial respiration remains one of the most important procedures ever introduced into the practice of medicine. Accordingly, it has played an unparalleled role in shaping the way we think about the pulmonary physiology and, in particular, about the pulmonary circulation. It is widely appreciated that, in addition to providing a beneficial exchange of respiratory gases at minimal metabolic cost to the patient during general anesthesia and during states of critical illness, this remarkably well-tolerated procedure introduces a host of “unphysiological” pulmonary as well as circulatory perturbations. The mechanism of these changes has been, and continues to be, the subject of numerous studies. It is proposed, however, that a key aspect of the artificial respiration tends to be overlooked, and yet it plays a pivotal role in the maintaining of the arterial circulation. As mentioned, the studies by Thompson [6], Manteuffel-Szoege [7], and recently by Skulec et al. [14] demonstrate the curious phenomenon of sustained blood movement which continues well beyond the time of cessation of heart’s action. To appreciate the nature of this phenomenon, it will be necessary to take a brief detour into the history of artificial respiration and, by way of illustration, examine the accounts of those who were the first to perform this radical intervention.
Historically, the quest of philosophers and physicians to fathom the unifying bond between the nature of the body and of the soul/spirit was intricately connected with respiration and the flow of blood. Respiration and the concept of biologic combustion was at the core of Galen’s system of medicine [58]. The process of respiration was regarded as a type of refined nutrition by which the lungs assimilate air (aer)5 and transform it into pneuma zotikon (zoe, vital spirit) in the blood. The blood undergoes further transformation, i.e., firing, in the heart where it combines with “innate warmth” (symphyton thermon), released in the left ventricle from the metabolic processes. It is then distributed as life-giving, arterial blood to the organs. In addition to intake of air, expansion of the thorax during inspiration also draws blood into the right heart where products of combustion are transformed into “soot” and exhaled as “smoke” from the lungs. A smaller portion of the blood seeps through the pores in the septum into the left ventricle where it combines with the inhaled pneuma. A final transformation of pneuma occurs in the ventricles of the brain (chorioid plexus), where it is converted into pneuma psychikon (spirit-soul). The latter serves two purposes: it directs the formative plan for the formation of the brain (and by extension, of the body) and serves as a nonmaterial substrate (faculty) for consciousness and sense perception in animals. In humans, it moreover upholds the faculty of rational thinking, the intellect (nous) [59].

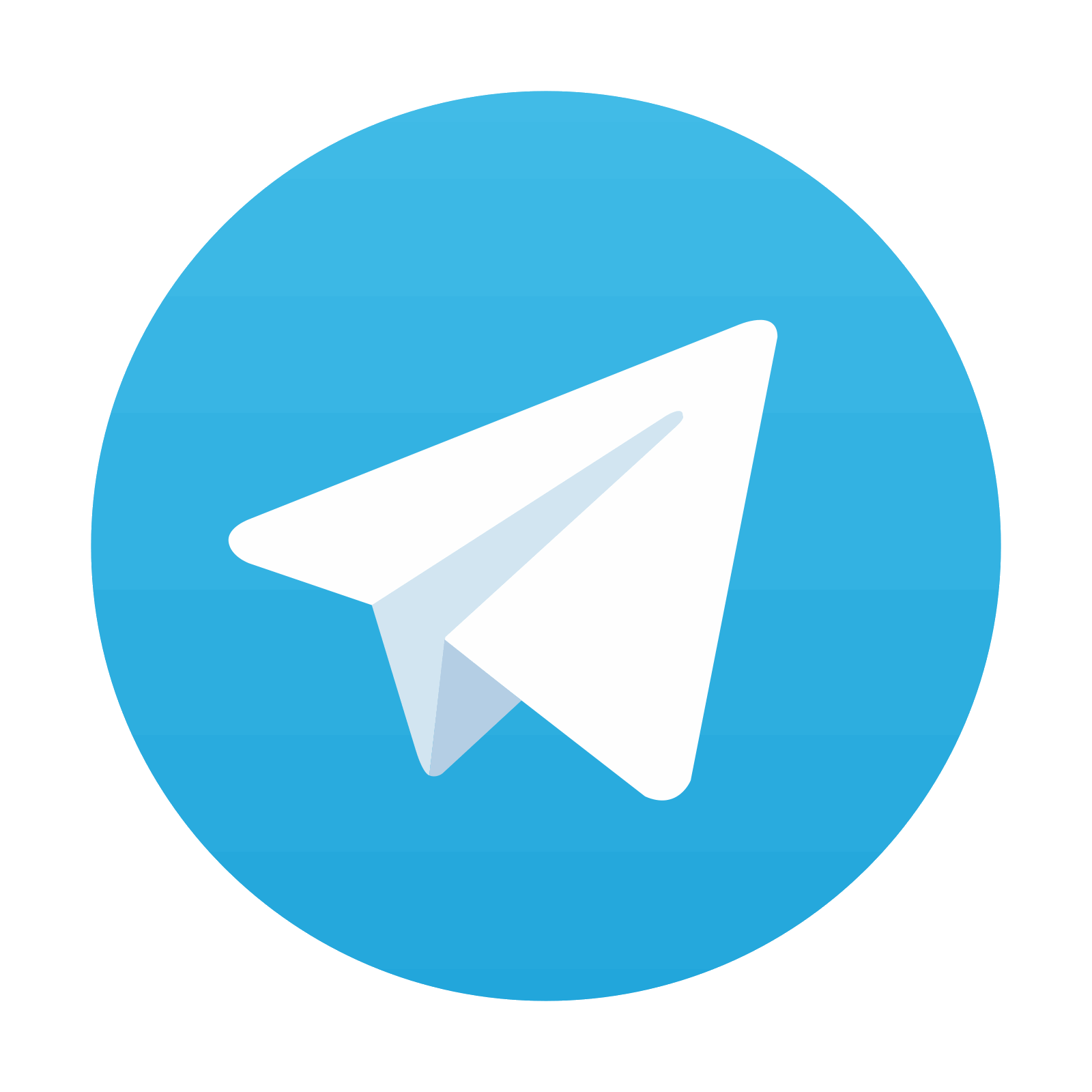
Stay updated, free articles. Join our Telegram channel
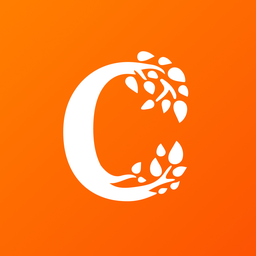
Full access? Get Clinical Tree
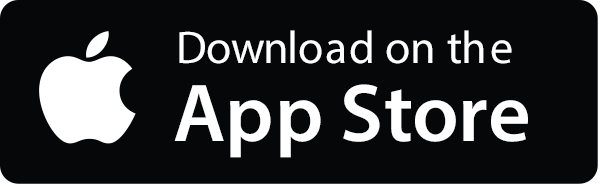
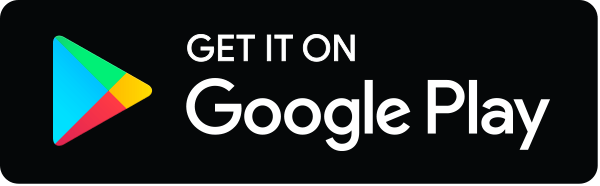