Outline
Role of Increased Adrenergic Drive in the Natural History of Heart Failure, 91
Adrenergic Receptor Pharmacology, 93
Altered β-Adrenergic Receptor Signal Transduction in the Failing Heart, 94
Molecular Basis of β-Adrenergic Receptor Signaling, 95
Regulation of β-Adrenergic Receptors Gene Expression, 96
Myopathic Potential of Individual Components of Adrenergic Receptor Pathways, 97
β 1 – and β 2 -Adrenergic Receptors, 98
α 1 -Adrenergic Receptors, 99
G-Proteins and Adenylate Cyclases, 99
G-Protein Receptor Kinases, 99
Adrenergic Receptor Polymorphisms and their Importance in Heart Failure Natural History or Therapeutics, 100
Noncatecholamine Ligands That Activate Myocardial β-Adrenergic Receptors, and their Role in Producing Dilated Cardiomyopathies, 101
Summary and Future Directions, 101
Normal and pathophysiological stresses result in increased myocardial demand that is met by a commensurate increase in cardiac adrenergic drive. To facilitate this increase, the neurotransmitter norepinephrine (NE) is released from adrenergic (sympathetic) nerve endings within the heart. Upon binding to β-adrenergic receptors (β-ARs), multiple signaling pathways are activated, resulting in increases in heart rate, force of contraction, and rate of relaxation. Increased adrenergic drive also results in metabolic adjustments (glucose vs. lipid) as well as in a multitude of changes in posttranslational modifications of proteins, gene expression patterns, and epigenetic modifications—notably to the acetylome and methylome. The immediate result of increased adrenergic drive is an increase in blood pressure and cardiac output. In the setting of acute heart failure, adrenergic activation occurs in response to hemodynamic overload and/or to an intrinsic reduction in pump function. However, in the setting of chronic decompensated heart failure, a response that is normally physiologic and transient is instead sustained in an attempt to maintain myocardial performance at a homeostatic level. Under these conditions, the initially beneficial adrenergic support mechanisms become manifestly maladaptive and contribute directly to the progressive natural history of heart failure.
In this chapter, the long-term consequences of sustained adrenergic activation in chronic heart failure (CHF) from reduced left ventricular ejection fraction (heart failure with reduced ejection fraction [HFrEF]), or CHF with pathologic eccentric remodeling and systolic dysfunction, will be reviewed. Particular emphasis will be placed on alterations in β-adrenergic signal transduction and gene expression patterns that occur in the context of the failing human heart, and the impact of these changes on myocardial disease progression. Adrenergic receptor pharmacology relevant to heart failure, as well as recent data from transgenic animals and model systems that demonstrate the myopathic potential of individual components of the adrenergic signaling cascade, will also be reviewed. These changes are likely to be applicable to CHF with preserved left ventricular ejection fraction (“HFpEF”); however, in human heart failure or animal models, HFpEF has not been investigated sufficiently to arrive at a firm conclusion. Therefore, in this discussion, CHF implies chronic HFrEF.
Role of Increased Adrenergic Drive in the Natural History of Heart Failure
In patients with CHF, the adrenergic nervous system is critical to the support of myocardial function. However, chronic activation contributes to progressive myocardial dys function and left ventricular pathological remodeling. Regardless of etiology, a decrease in myocardial function results in activation of afferent signaling from baroreceptors and chemoreceptors to the central nervous system, such that efferent cardiac adrenergic nerves release more NE, activating β-ARs, increasing heart rate and, depending on the degree of functional reserve, increasing myocardial contractility. The increases in heart rate and contractility favorably affect both systolic and diastolic function, thereby increasing cardiac output. As heart failure worsens, adrenergic drive continues to increase in an attempt to compensate for the progressive loss of cardiac function ( Fig. 6.1 ). However, long-term exposure to high concentrations of NE has marked adverse effects on myocardial and cardiac myocyte biology ( Fig. 6.2 ). Conversely, inhibitors of adrenergic signaling that have demonstrated favorable effects on heart failure natural history include β-blocking agents and inhibitors of the renin–angiotensin–aldosterone system, which indirectly lower adrenergic drive. There is extensive clinical trials literature supporting the use of these classes of agents as well as definitive guidelines (American Heart Association/American College of Cardiology [AHA/ACC]) for their optimal administration (see Chapter 37 ).


Although the “cardiotoxic” effects of catecholamines, in particular NE, have been recognized for more than a century, we have only recently begun to understand the detailed molecular mechanisms by which sustained increases in cardiac adrenergic drive adversely affect myocardial biology and structure/function phenotype. Treatment of isolated cardiac myocytes with concentrations of NE equivalent to those in the failing human heart cause dramatic changes in cell morphology and, within a matter of days, up to a 60% loss of myocyte viability. This effect is mimicked by exposing myocytes to the nonselective β-agonist isoproterenol, an effect that is inhibited pharmacologically by the β-blocker, propranolol, suggesting that the acute toxic effects of NE are mediated almost exclusively through β-ARs rather than through α-ARs. As described below, signaling via the β 1 -AR is generally considered to be more biologically harmful than via the β 2 -AR. This finding is supported by clinical trial data, where differences in β 1 -AR blocking doses and enrollment criteria are considered ; further, β 1 -AR selective blockade appears equally efficacious as treatment with nonselective β-blockers, meaning that β 1 -AR signaling is the key general mechanism mediating reversible pathobiologic effects in the failing heart.
As stated above, elevated plasma concentrations of NE reflecting overflow from adrenergically activated organs including the heart are a well-recognized biomarker of CHF. However, as adrenergic activation persists, cardiac NE stores become depleted. Ultimately, other tissue sources of catecholamines (i.e., the adrenal medulla) are stimulated, leading to generalized adrenergic activation and spillover of cardiac derived NE into the systemic circulation. As systemic or cardiac NE levels increase, so does mortality in patients with heart failure. These observations have been validated further by evidence from numerous clinical trials of β-blocker therapy, demonstrating reverse remodeling (improved systolic function and decreased left ventricular volumes, typically measured as the ejection fraction) and improved survival in patients with heart failure who receive β-blocker therapy, long term.
Interestingly, in marked contrast to adults with HFrEF, β-blocker therapy does not appear to be particularly efficacious in pediatric heart failure patients. Although the findings are somewhat anecdotal, pediatric patients with systolic heart failure appear to respond more favorably than adults to type 3 phosphodiesterase (PDE) inhibitors. Underlying this difference are markedly different gene expression patterns and adrenergic receptor biology in adult versus pediatric populations.
A recent realization is that insufficient adrenergic drive in individuals with CHF can also increase mortality. In contrast to β-blocking agents, sympatholytic agents (agents that lower adrenergic activity) can actually increase mortality, presumably via excessive withdrawal of adrenergic support to the failing heart. In contrast, β-blocking agents are pharmacologically reversible mass-action agents, and in their presence adrenergic support to the failing heart can be accessed if needed simply by increasing cardiac adrenergic drive. One β-blocker in clinical development, bucindolol, is both a nonselective β-blocker and a mild sympatholytic agent. When NE lowering from bucindolol is mild, the result is an enhancement of efficacy. However, in some patients, the degree of sympatholysis produced by bucindolol can be excessive, obviating any benefit realized from the β-blockade. It is now understood that the sympatholytic effects of bucindolol are under control of prejunctional α 2C adrenergic receptors, the implication being that exaggerated/adverse NE lowering can be avoided by not treating patients who have a loss of function α 2C polymorphism in combination with a decreased function β 1 -AR genetic variant (see below).
Historical studies suggested that one underlying mechanism of NE toxicity involves cyclic adenosine monophosphate (cAMP)-mediated increases in intracellular calcium. Increased formation of the second messenger cAMP leads to activation of protein kinase A (PKA), which in turn phosphorylates a variety of target proteins involved in regulating intracellular calcium, including key targets such as phospholamban (PLB), L-type calcium channels (LTCCs, CaV 1.2 ), and ryanodine receptors (RyR2). Enhanced phosphorylation of calcium channels leads to an increased flux of extracellular calcium into the cell, activating downstream effectors including proteases, kinases, and phosphatases. Activation of the β 1 -AR subtype also appears to promote increased calcium influx via cAMP-independent mechanisms. Importantly, β 1 -ARs can be cAMP-independent activators of CaMKII, a signaling pathway known to have particularly adverse effects on cardiac myocytes. Regardless of the exact mechanism(s) invoked, excessive β-AR stimulation results in oxidative stress and calcium overload, conditions promoting cell death through a number of mechanisms, including necrosis and apoptosis. Long-term adrenergic activation has also been shown to cause changes in the gene expression profile of cardiac myocytes, the general response being activation of the so-called fetal gene program that is under β 1 -adrenergic control via membership in a larger gene signaling network. As discussed in Chapter 1 , the fetal gene program, which is a molecular surrogate for hypertrophy/heart failure, includes downregulation in the gene expression of the fast-contracting α-isoform of myosin heavy chain (MHC) (MYH6) and SR Ca 2+ ATPase (ATP2A2) , upregulation of the slow contracting β-MHC isoform (MYH7) , and upregulation of ANP (NPPA) and BNP (NPPB) .
In the failing heart, β-ARs and certain downstream PKA-dependent effectors in the β-adrenergic signaling cascade undergo agonist-mediated, time-dependent downregulation and desensitization, presumably in an attempt to withdraw the heart from an excess of deleterious signaling. These changes result in a marked attenuation of the failing heart’s ability to respond to either endogenous or exogenous catecholamines. Consequently, the amount of cAMP generated in response to a given amount of adrenergic stimulation can be significantly decreased. Evidence has been provided through experiments using isolated, denervated preparations of human heart tissue revealing that failing hearts have a reduced cAMP content compared to nonfailing controls. Extending this observation to the clinical standpoint, reduced responsiveness to catecholamines translates into a decrease in myocardial reserve, which is most commonly manifested as an impaired ability to exercise and means less ability to respond to any form of circulatory stress. In contrast, PKA-independent signaling pathways, such as CaMKII, may undergo upregulation.
In summary, the role of increased adrenergic drive in the natural history of heart failure is a seemingly a paradoxical one. On the one hand, adrenergic activation is essential to meeting the demands of increased physiological and pathological stress in the failing heart; on the other hand, chronic adrenergic stimulation is a major contributor to progressive myocardial dysfunction and remodeling that characterizes heart failure. However, from an evolutionary biology standpoint, this situation is entirely plausible; adrenergic mechanisms have evolved to allow reproductive-age individuals to cope with and escape from highly stressful short-term situations; they are not particularly well suited to chronic support of the failing heart that typically occurs beyond the reproductive years.
Adrenergic Receptor Pharmacology
In the 1940s, Ahlquist recognized that adrenergic receptors could be subdivided into two major classes, α- and β-ARs ( Fig. 6.3 ), both of which serve as binding sites for the endogenous catecholamines, epinephrine and NE. Almost 20 years later, Lands and colleagues, using rank orders of agonists, proposed that β-ARs could be divided into β 1 – and β 2 -AR subtypes. A few years later, use of selective β 1 – and other β-AR antagonists formalized this classification and expanded it to include an additional β-AR subtype, later named the β 3 -AR. Building on this basic pharmacology was work in the 1970s and 1980s centered on direct identification of receptors by radioligand binding and the biochemistry of signaling pathways. Based on the groundbreaking peptide biochemistry work of Khorana and colleagues on rhodopsin, subsequent work by Lefkowitz and colleagues described the molecular cloning of a number of G-protein coupled receptors (GPCRs) including α 1 -, α 2 -ARs, and the β 1 – and β 2 -ARs. Perhaps more than any other previous advance in the field, this knowledge permitted the analysis of adrenergic receptor biology in exquisite detail and allowed for an appreciation of the true complexity of adrenergic receptor signaling, including the definition of a multitude of gene regulatory motifs and the recognition of highly organized multi-protein signaling complexes. Ultimately, the combined work of Lefkowitz and Kobilka on GPCR pharmacology, biochemistry, and molecular biology, as well as Kobilka’s subsequent work on GPCR structural biology, resulted in their being awarded the Nobel Prize in Chemistry in 2012.

Relevant to the human heart, there are two major subclasses of α-ARs, α 1 – and α 2 -ARs, of which there are several subtypes (see Fig. 6.3 ). These α 1 -AR subtypes are coupled to the Gq/G11-family of G-proteins, linked to stimulation of phospholipase C (PLC), which in turn promotes phosphoinositide (PIP 2 ) turnover. The two primary products of this reaction are inositol triphosphate (IP 3 ), which stimulates the release of intracellular calcium, and diacylglycerol (DAG), which stimulates protein kinase-C (PKC) activity. Increases in intracellular calcium and PKC-mediated phosphorylation of target proteins both activate signaling pathways and gene expression patterns which, depending on the PKC subtype, result in a hypertrophic myocardial phenotype as well as vasoconstriction. Examples of other Gq-coupled receptors whose stimulation also results in a hypertrophic phenotype are the angiotensin II AT1 and endothelin-1 receptors. Each of these receptor pathways represents pharmacological targets central to the management of heart failure and/or its precursors, such as systemic or pulmonary hypertension.
In contrast to α 1 -, α 2 -ARs are generally described as inhibitory presynaptic or prejunctional neuronal receptors functioning to suppress the release of NE from the synapse. The potential importance of this function in the setting of heart failure has been emphasized in a study by Small and colleagues, wherein a naturally occurring “loss of function” polymorphism of the α2C-AR, when present in combination with a “gain of function” polymorphism of the β 1 -AR, produced a significantly increased risk of heart failure in certain populations. These and other β-AR polymorphisms, and their relevance to CHF, are discussed in greater detail below.
β-ARs are also subdivided, with three distinct receptor subtypes encoded by separate genes β 1 – (ADRB1) , β 2 – (ADRB2) , and β 3 – (ADRB3) . β 1 – and β 2 -ARs were first described by agonist or antagonist pharmacologic responses, and then by molecular cloning. In contrast, β 3 -ARs were first definitively identified by molecular cloning after being classified as beyond the β 1 , β 2 paradigm or “atypical” in various organs, as determined by pharmacologic response. β 3 -ARs are generally associated with regulation of metabolic rate, modulation of nitric oxide (NO) formation, gut and urinary tract smooth muscle relaxation and, to a lesser degree, with changes in cardiac contractility. β 3 receptors are expressed at low abundance but are coupled via a nitric oxide synthase (NOS)/NO/soluble guanylate cyclase/cGMP mechanism to a negative inotropic response in human ventricular myocardium, where they may play a cardioprotective role. In contrast, β 1 – and β 2 -ARs have the clear functional role of increasing myocardial performance, and both are expressed at a substantial abundance in human ventricular myocardium with β 1 -AR density greater than β 2 .
β 1 – and β 2 -ARs, respond equally well to the full agonist isoproterenol, and are distinguished primarily by their affinity for NE. β 1 -ARs have 10- to 30-fold greater affinity for NE than β 2 -ARs. An even greater degree of distinction is achieved through the relative affinities of selective antagonists. In the context of heart failure, the most clinically relevant compounds differentiating between β 1 – and β 2 -ARs are the β 1 -AR selective antagonists metoprolol, bisoprolol, and nebivolol. In contrast, β 3 -ARs are characterized by their relatively low affinity for propranolol, by the selective β 1 -antagonist CGP 20712a, or by their response to β 3 selective agonists such as BRL 37344 42 or CL316243. Two β-blocking agents in use or development, nebivolol and bucindolol, have β 3 -agonist activity that may contribute to their vasodilator properties or possibly to their myocardial effects.
Altered β -Adrenergic Receptor Signal Transduction in the Failing Heart
As manifest in heart failure, chronic heightened adrenergic activation ultimately results in diminished β-AR-mediated signaling as a consequence of decreased in β 1 -AR gene expression (mRNA and protein expression), uncoupling of both β 1 – and β 2 -ARs secondary to receptor phosphorylation from βARK1 (GRK2) or other kinases, and to increases in the inhibitory G protein(Gαi) ( Fig. 6.4 ). In nonfailing, adult human ventricles, β 1 -ARs constitute approximately 70% to 80% of all β-ARs. However, in individuals with heart failure the β 1 -AR is selectively downregulated, resulting in an ∼60:40 ratio of β 1 – to β 2 -ARs.

Phosphorylation of β-ARs occurs by the G-protein receptor kinase (GRK) family, specifically GRK2, which phosphorylates both β 1 – and β 2 receptor subtypes in an agonist occupancy-dependent manner, and GRK5, a kinase of considerable importance linking β 1 -ARs to epidermal growth factor receptor (EGFR) transactivation and cardioprotective effects, but also to CaMKII signaling, and β 2 -ARs to Src and MAP kinase (extracellular signal-regulated kinase [ERK]) signaling. As initially described, β-ARs also can be phosphorylated and desensitized by other kinases including PKA and PKC. Therefore in failing ventricular myocardium, both β 1 – and β 2 -receptor subtypes undergo desensitization, with β 1 -ARs exhibiting downregulation, and both β 1 – and β 2 -ARs uncoupling from PKA signaling. Similar results have been obtained in the in vivo setting with intravenous administration of dobutamine, demonstrating marked blunting of contractility in the failing human heart compared to no decrement in function with calcium infusion.
The major consequence of β-AR downregulation and desensitization is that for a given level of adrenergic activation, less of the second messenger cAMP is produced. In turn, decreased formation of cAMP leads to diminished activation of PKA. However, decreased PKA activity does not necessarily translate into decreased phosphorylation of all putative PKA substrates. Related to the above, although PKA activity and PLB phosphorylation are decreased in heart failure, cardiac RyR2s have been shown to be hyperphosphorylated. The net phosphorylation “state” of the RyR and PLB, and thus their activities, is due, at least in part, to their being in separate subcellular A-kinase anchoring protein-scaffolded microdomains. In these discreet microdomains different PDEs control cAMP levels and PKA activation; phosphatase species and activities may also differ. Specifically, in the failing heart there is evidence for the downregulation of phosphatases PP1 and PP2A that are associated with RyR2, as well as to changes in PKA activity. Alternatively, recent studies have shown that RyR2 can be phosphorylated by CaMKII, inducing SR calcium leakiness and engendering arrhythmias, an effect that appears to be mediated by cardiac β 1 -ARs and the “exchange protein directly activated by cAMP-2,” EPAC2. Interestingly, a recent paper by Pereira and colleagues, showed that the EPAC/CaMKII-mediated phosphorylation of RyR2 occurs through a pathway that includes AKT (also known as protein kinase B) and NOS1. The authors showed that increased cAMP levels activate EPAC, which in turn activates AKT resulting in NOS1 activation. NOS1 promotes S-nitrosylation of CaMKII resulting in phosphorylation of RyR2 and increased leakiness.
In contrast to the hyperphosphorylation of RyR2, PKA-mediated phosphorylation of PLB appears to be decreased in heart failure, which is presumably due to reduced cAMP levels in the SERCA II-PLB microdomain and to the activation of the phosphatase PP1. PP1 is regulated by protein phosphatase inhibitor I, PPI-1, a protein whose abundance is itself downregulated in heart failure. The reduced PLB phosphorylation results in a greater inhibition of SR Ca 2+ ATPase (SERCA II), resulting in an impaired ability of the heart to both relax and contract. In contrast, hyperphosphorylation of RyR2 results in defective channel function during excitation-contraction coupling. Together, these changes result in decreased contractility in response to adrenergic stimulation and a propensity for developing arrhythmias. Unfortunately, however, the relationship between RyR2 phosphorylation state and its biological relevance is not as clear as might be desired, as articulated in a recent set of “controversies” articles.
Substantial evidence from model systems points to significant differences between β 1 – and β 2 -ARs and their ability to stimulate apoptosis in cardiac myocytes with stimulation of the β 1 – but not the β 2 -AR pathway resulting in an increased rate of cardiomyocyte apoptosis. Disruption of β 2 -AR coupling to Gi by treatment with pertussis toxin can abrogate the potential protective, anti-apoptotic signaling pathways rendering the β 2 -AR pro-apoptotic. Chesley and colleagues, have provided evidence to support the supposition that the anti-apoptotic effects of β 2 -AR are mediated in part by the stimulation of the PI3K and the Akt-PK-D pathways.
In addition to the mechanisms described above, the pro-apoptotic effect of the β 1 -AR pathway also may be due to increased CaMKII activity. Zhu and colleagues, have shown that β 1 -AR stimulation results in myocyte apoptosis, which is independent of PKA activation but is dependent on CaMKII, suggesting that β 1 -AR stimulation may selectively result in CaMKII activation independently. These studies demonstrate that inhibition of CaMKII, LTCC phosphorylation, or buffering of intracellular Ca 2+ results in an attenuation of β 1 -AR mediated apoptosis; conversely, overexpression of CaMKIIδ c induces apoptosis. In addition, Yoo and colleagues, using transgenic mice with knockouts of β-AR subtypes, have demonstrated that post-infarct, isoproterenol-mediated increases in CaMKII signaling and apoptosis are dependent exclusively on the β 1 -AR. However, recent data from Dewenter and colleagues indicate that CaMKII signaling persists despite the use of β-blockers in both experimental and human heart failure, arguing for the development and use of selective CaMKII inhibitors in heart failure. This conclusion is supported by the findings of Kreusser and colleagues, indicating that in a mouse transverse aortic constriction (TAC) model, double knockout of CaMKIIδ/γ progression of cardiac dysfunction and deposition of fibrosis is attenuated.
Excessive β 1 -AR signaling also activates the fetal gene program ( see also Chapter 1 ). In this context, a change in MHC isoform expression favoring the low(er) ATPase activity β isoform would be expected to decrease contractile function, as would the downregulation in SERCA II, another adult gene that is considered part of the adult-fetal program. Although the signaling cascades responsible for this activation are complex, CaMKII appears to play a prominent role, and the altered expression of so-called fetal and adult genes appears mediated by β 1 – and not β 2 -AR stimulation. CaMKII activity is upregulated in the failing human heart, suggesting that despite the desensitization that occurs in more proximal β 1 -receptor signaling steps, adverse signaling through CaMKII may be maintained or even enhanced ( see also Chapter 1 ).
As described above, CaMKII is involved in various pathologic processes in heart failure including apoptosis, arrhythmias and changes in gene expression. To gain a better understanding of the role of CaMKII in pathologic cardiac disease, knockout mouse models of CaMKII and a transgenic model overexpressing a CaMKII peptide inhibitor have been generated. Mice expressing the inhibitory peptide are resistant to pathological changes related to β-AR stimulation, and do not exhibit decreases in fractional shortening, increases in heart/body weight or decreases in left ventricular internal diameter. Different results are obtained in knockout mouse model studies. In response to TAC in 6-week-old animals, knockout of CaMKIIδ blunted fibrosis and reduced heart/body weight ratio, myocyte area, and pathologic changes in gene expression. In an 8-week TAC model, CaMKIIδ downregulation did not prevent the development of pathologic hypertrophy, but blunted the transition to heart failure, including chamber dilation, ventricular dysfunction, lung edema, cardiac fibrosis, and apoptosis. However, a 6-week-old CaMKII knockout mouse model of severe thoracic aortic banding showed improved contractility, increased myofilament sensitivity to Ca 2+ , decreased apoptosis but increased fibrosis. However, no improvement in ejection fraction/fractional shortening was observed. The authors also showed that knockout of CaMKII in the setting of severe heart failure may be detrimental due to reduced efficiency of β-AR regulation of contraction and exacerbation of diastolic dysfunction. These studies suggest that although CaMKII is likely to be primarily detrimental, it may also be important for β-AR regulation of contractile function in the failing heart.
In contrast to the general desensitization phenomena exhibited by β 1 – and β 2 -ARs in failing human ventricular myocardium, β 3 -ARs exhibit upregulation. Since the β 3 -AR knockout mouse undergoes excessive remodeling in response to pressure overload, increased expression of β 3 -ARs in the failing human heart may be a salutary adaptive mechanism.
Molecular Basis of β -Adrenergic Receptor Signaling
Canonically, β 1 – and the β 2 -ARs couple to stimulatory G-proteins, Gs, which are composed of three subunits, an α-subunit, and a βγ-heterodimeric subunit, Fig. 6.5 . Both the α and βγ subunits are competent to signal independently Gαs is a member of the much larger class of G-protein α-subunits, of which there are ∼20 subtypes. Their heterogeneity is a central basis of specificity for GPCR signaling, a subject reviewed in detail elsewhere. Additional layers of signaling diversity are afforded by combinatorial permutations of various βγ-heterodimeric subunits (5 β-subtypes and 11 γ subtypes) with the various α-subunits.

As a result of agonist stimulation, G-protein α and βγ subunits dissociate with the free αs-subunit acting to stimulate adenylate cyclases (AC), for which there are multiple isoforms. In cardiac tissues, the most abundant isoforms are AC V and VI with the activities of each being feedback inhibited by increases in cytosolic calcium. In turn, adenylate cyclase stimulation increases production of cAMP that, in turn, binds to the regulatory subunits of PKA. Binding causes the dissociation of the regulatory and catalytic subunits of PKA, with the catalytic subunits proceeding to phosphorylate consensus serine (S) and threonine (T) residues on a broad spectrum of intracellular target proteins. In a number of tissues, including cardiac, PKA and its targets are in close approximation because of A-kinase anchoring proteins (AKAPs). An association between the β 2 -AR and AKAP79 has been described. Additionally, there is recent clarity as to the scaffolding proteins that interact with β 1 -ARs. Thus, unique interactions between β 1 – and β 2 -ARs and specific AKAPs and/or other anchoring proteins support the “micro-domain” concept of receptor subtype-specific signaling, discussed in greater detail below.
Via the “canonical” Gαs/adenylyl cyclase pathway, β-AR stimulation results in the phosphorylation by PKA of a number of proteins including (LTCC, CaV 1.2 ) and PLB, the primary modulator of the sarcoplasmic reticulum (SR)-associated ATP-dependent calcium pump, SERCA II, as well as modulatory proteins associated with regulation of the contractile apparatus, for example, troponin I (TnI). More directly, however, PKA phosphorylates β-ARs, as well as other GPCRs, resulting in partial uncoupling and desensitization of receptors to further agonist stimulation. Changes in the phosphorylation state of calcium-handling proteins results in increased [Ca ++ ]i and enhanced rates of myocardial contraction and relaxation. However, the increase in intracellular calcium has a number of other effects beyond enhanced contractility, including the activation of a number of calcium-sensitive enzymes, for example, Ca ++ /calmodulin and calcineurin (phosphatase PP2B). On a more global level, changes in [Ca ++ ]i can result in profound changes in gene expression patterns.
It has been known for some time that β 1 – and β 2 -ARs differ in their efficiency of coupling to adenylate cyclase and to cAMP production, with the β 2 -AR subtype being more efficiently coupled to cAMP production than is the β 1 -AR. In trabeculae isolated from the human heart, selective stimulation of contraction by either receptor pathway generally correlates well with receptor abundance. However, the fact that the β 2 -AR produces more cAMP per unit of stimulus does not necessarily translate into a greater inotropic potential for β 2 -ARs. Rather, these data favor an argument that there is distinct intracellular compartmentalization of cAMP pools produced by β-ARs, with the existence of discrete microdomains of high cAMP concentration in cardiomyocytes. Specifically, in neonatal cardiomyocytes, high concentrations of cAMP are found in the proximity of T tubules and the junctional SR. An argument is also made that diffusion of cAMP outside of these microdomains is quickly curtailed by the action of PDEs. Support for this notion is the finding that there appears to be close coordination between agonist-stimulated recruitment of PDEs to the β 2 -AR, a process facilitated by interaction with β-ARs, and the attenuation of signaling. Recent data indicate that the differential signaling and architecture of β 1 – and β 2 -ARs appears to be defined, in part, by a differential association with PDEs, with PDE4 activity preferentially targeting cAMP produced by the β 1 -AR, whereas cAMP produced by the β 2 -AR being targeted by multiple subtypes of PDEs. Perhaps counterintuitive to the highly localized responses of β-ARs being dictated by scaffolding proteins is the recent study by Nikolaev and colleagues, demonstrated in transgenic mice that isoproterenol-mediated increases in cAMP in cardiac tissues leads to “far-reaching” cAMP signals for the β 1 -AR, whereas β 2 -AR generated cAMP appears to remain locally confined. An excellent recent study detailing the compartmentalization of β-AR signaling, the interplay of PDEs, phosphatases, and subtype specific spaciotemporal patterning has recently been published by Xiang.
A very recent study delineates further cAMP and β-AR compartmentalization. Interestingly, in murine models, β 2 -ARs and cAMP demonstrate anatomical variation such that at the apex of the heart β 2 -ARs uniquely modulate increased contractility. This appears to be primarily due to variations in the size and organization of T-tubules and caveolae impacting microdomains.
It is clear that both the β 1 -AR and the β 2 -AR pathways couple to signaling pathways beyond the “traditional” Gαs/adenylyl cyclase pathway. Perhaps the best example of this diversity of signaling is the finding that the β 2 -AR can interact with the inhibitory G-protein, Gαi. This is of interest in the setting of CHF, for two reasons. As described earlier, increased circulating NE concentrations result in chronic activation of β-ARs. This, in turn, results in increased βARK activity and an increased phosphorylation state of β-ARs resulting ultimately in the uncoupling of the receptor from the stimulatory Gαs signaling pathway. It is this phosphorylated form of the β 2 -AR that has an increased propensity to couple to the inhibitory Gαi pathway and its downstream effectors, including inhibition of AC and activation of MAPKs. Amplifying this effect is the finding that the abundance of Gαi proteins is significantly elevated in the failing human heart. Thus, the increased phosphorylation state of the β 2 -AR and the increased abundance of Gαi protein both facilitate increased trafficking of β 2 -AR signaling via Gαi-associated pathways. The ramifications of this are potentially significant, particularly in regard to protective, anti-apoptotic effects as well as to myocardial growth regulatory effects.
The β 1 -AR, although thought to be less “promiscuous” than the β 2 -AR in its ability to couple to pathways other than to Gαs, nonetheless does exhibit signaling via additional pathways, as noted above, to the CaMKII pathway. However, recent data from Wang and colleagues support the conclusion that the β 1 -AR can indeed couple to Gαi, an effect uniquely promoted by the β-AR antagonist, carvedilol. In fact, Gαi appears to be required for β 1 -AR β-arrestin-biased signaling for both EGFR transactivation and for MAPK signaling. However, unlike β 2 -AR Gαi-mediated ERK activation, carvedilol-induced β 1 -AR/Gαi MAPK signaling does not appear to require Gβγ nor GRK/PKA receptor phosphorylation. As noted above, current published evidence supports the finding that carvedilol’s induction of β 1 -AR/Gαi interaction is unique; it will be of future interest to determine whether or not nebivolol, also a β-arrestin-biased agonist, has any requirement for Gαi.
At least in part, β 1 -AR extra-Gαs coupling, much like that of the β 2 -AR, appears to be facilitated by the specificity of the carboxy-terminus PDZ (PSD-95/Dlg/ZO-1) domain of the receptor peptide, a domain that drives the interaction with proteins containing specific PDZ recognition motifs. An excellent review on the general topic of regulation of β-AR signaling by scaffolding proteins has been published by Hall and Lefkowitz. Examples of β 1 -AR interacting proteins are PSD-95, MAGI-2, the endophilins (SH3p4/p8/p13), and CNrasGEF, all of which appear to have unique downstream roles. The association of the β 1 -AR with PSD-95, which is a neuronal-associated protein, distinctly places the β 1 -AR in the proximity of the synapse, a location perhaps driven by adrenergic innervation. Here, PSD-95 appears to inhibit the internalization of the β 1 -AR, an effect that is modulated by the kinase GRK5. Conversely, interaction of the β 1 -AR with endophilins appears to negatively modulate Γαs coupling and to promote β 1 -AR internalization. A more detailed picture of the β 1 -AR SAP97-AKAP79/150 scaffold at its PSD-95/DLG/ZO1 motif has been demonstrated to be necessary for both PKA mediated phosphorylation of the β 1 -AR as well as for receptor trafficking. This is in contrast to the findings describing the association of the β 1 -AR with CNrasGEF (PDZ-GEF1), an effector molecule that activates Ras, and thus growth pathways. In this case, the mechanism of Ras activation is rather unique given that it appears to be via Gαs rather than the well-documented Gβγ signaling pathway.
Additional evidence detailing the importance of PDZ-mediated interactions has been described by Xiang and colleagues. Specifically, mutation of the PDZ motif by disruption of the carboxy-terminus renders the β 1 -AR significantly more sensitive to agonist-mediated cell surface downregulation. Further, deletion of C-terminal amino acids renders the β 1 -AR more β 2 -AR-like in that it becomes capable of interacting with Gi. Thus, even though the β 1 -AR and β 2 -AR both contain PDZ motifs, the interacting proteins that each couples to are unique and serve as an important basis of different regulatory and signaling properties. To expand this concept, the β 2 -AR, has been shown to modulate signaling independent of its interactions with G-proteins. For example, the β 2 -AR appears to couple to at least three other pathways: (1) NHERF, which in turn regulates the function of the Na + /H + exchanger ; (2) to a non-PKA-dependent interaction with L-type calcium channels ; and (3) coupling to the phosphatidylinositol 3′-kinase pathway (PI3K), a pathway associated with the inhibition of apoptosis, and to the PI3K-PTEN pathway, which is relevant to the regulation of both myocardial contractility and cell size and shape.
As alluded to above, β-AR stimulation results in the dissociation of the stimulatory G-protein, Gs, into α and βγ subunits, each of which are signaling entities. A major function of the βγ heterodimer is to recruit βARKs in proximity to the receptor. By virtue of the lipid-modified (isoprenylation) of the γ subunit, which promotes localization of the βγ subunit to the sarcolemmal membrane, the heterodimer is in position to orchestrate the co-localization of the β-AR with βARK. Protein/protein interaction of the βγ subunit with βARK occurs via a pleckstrin homology (PH) domain. In this way agonist-dependent, βARK-mediated phosphorylation of β-ARs serves as an important mode of β-AR desensitization. In addition to playing a scaffolding role, βγ subunits are also signaling molecules in their own right. Downstream targets of signaling include subsets of adenylyl cyclases, PI3K, K + channels, and Ras/Raf/MEK/MAPK pathway(s) (see Fig. 6.5 ).
Recently, significant attention has been paid to the specifics of β-AR localization and receptor trafficking. Differential targeting of β-AR subtypes to specific intracellular compartments has been described. Of note is the apparent caveolar co-localization of the β 2 -ARs with the inhibitory protein, Gαi, as well as with PKA subunits and with GRK2. What is now becoming clear is that the localization of the β 2 -AR to the caveolar compartment is an essential component of its signaling capabilities. In contrast, β 1 -ARs, as well as a number of other proteins including Gαs, and ACs V and VI, are not specifically associated with caveolae; instead, these proteins have a more generalized distribution.
An important and newly emergent concept of β-AR signaling is that of the connection between β 1 -AR signaling with the cardioprotective effects of the EGFR pathway. Work from Rockman and colleagues has shown that GRK5/6 mediated phosphorylation of the β 1 -AR targets the association of β-arrestin to the receptor, which, in addition to activating MAP kinases, facilitates the activation of matrix metalloproteinases causing the release of heparin-bound EGF, which then activates the EGFR. Activation of the EGFR leads to activation of Ras/Raf/ERK/Akt and subsequent anti-apoptotic, cardioprotective effects (see Fig. 6.5 ).
Recent evidence indicated that the differentiation of β 1 – versus β 2 -AR signaling also extends to the cardiac progenitor cells (CPCs) and their differentiation and survival. Interestingly, β-AR stimulation appears to promote the differentiation of CPCs via β 2 -ARs. However, once these cells become committed to a myocyte lineage, they begin to express β 1 -ARs. Counteracting that progression, continued β-AR stimulation via β 1 -AR leads to a loss of CPCs.
Regulation of β -Adrenergic Receptors Gene Expression
Genes encoding β-ARs are regulated at virtually every level, including transcriptional, posttranscriptional/pretranslational, translational, and posttranslational. Much like proto-oncogenes and cytokines, β-ARs can profoundly affect a number of cellular functions affecting cell viability—thus the need for stringent, rapid, and redundant regulatory mechanisms. β-AR expression, at the levels of both mRNA and protein, is the result of a number of independently regulated processes.
At the level of transcriptional regulation, both positive and negative transcriptional regulatory elements exist within the 5′-untranslated regions (UTRs) of β-ARs. Cell culture model systems have provided evidence regarding the effects of a number of transcriptional regulatory pathways starting with the most obvious, that of the direct effects of β-AR stimulation. Increases in cAMP can lead to a transient upregulation of β-AR mRNA via a cAMP-responsive element (CRE)-mediated transcriptional effect. However, it is recognized that in heart failure, at least for the β 1 -AR, mRNA and protein are unequivocally downregulated. Therefore, CRE-mediated upregulatory effects are unlikely to be important in the chronic setting. Conversely, β-AR genes have the potential to be negatively regulated by the transcriptional suppressor, ICER (inducible cAMP early repressor). Evidence points to a role for ICER in a pathophysiological feedback loop with its upregulation causing a persistent downregulation of PDE3A—a finding associated with increased isoproterenol-mediated cardiomyocyte apoptosis. However, others have not found a downregulation in PDE3 in the failing human heart.
In the cardiac context, a number of other transcriptional regulators of β-AR genes are also known to be important. Most notably, transcriptional regulation of β-AR genes by glucocorticoid and thyroid hormone-responsive elements can have significant effects via their respective consensus DNA binding elements (e.g., glucocorticoid response elements [GREs] and thyroid hormone response elements [TREs]). In general, glucocorticoids have been associated with causing an upregulation of β 2 -ARs and a reciprocal downregulation of β 1 -Ars, whereas thyroid hormone has been uniformly associated with upregulation of β-AR expression.
For β-ARs expressed in myocardial tissues, a concordant relationship between mRNA and protein appears to be well preserved. Thus, mechanisms regulating β-AR mRNA abundance likely significantly impact β-AR protein expression. To this end, nucleic acid motifs, known as A + U-rich elements (AREs), found within the 3′UTRs of several β-ARs, are known to be the target for a number of mRNA binding proteins associate with changes in mRNA turnover. Previous evidence has confirmed a relationship between agonist-mediated stimulation of β-ARs, the production of cAMP, and the destabilization of β-AR mRNA resulting in reduced mRNA and ultimately protein abundance. However, additional evidence points to the role of MAPK pathways as critical regulators of the stability of ARE-containing mRNAs, including those encoding β-ARs. In particular, several proteins associated with mRNA changes in mRNA turnover have been demonstrated to bind to β-AR AREs. These include AUF1/hnRNP D, which is associated with increased mRNA turnover ; HuR, which is associated with the stabilization of numerous ARE-containing mRNAs ; KSRP, which increases turnover of ARE-containing mRNAs, drives muscle cell differentiation, and modulates Wnt/β-catenin and PI3K/AKT signaling ; and tristetraprolin (TTP), which is associated with turnover of tumor necrosis factor α (TNFα) mRNA. How each of these proteins specifically affects β-AR mRNA turnover in the myocardial tissues remains to be explored. However, given the evidence that the homozygous deletion of TTP in transgenic mice results in a dramatic upregulation of TNFα and a subsequent cardiomyopathy, there is little doubt that these mechanisms are in play.
Other means of posttranscriptional regulation include modulation of translational efficiency that can be controlled by RNA-binding proteins and by microRNAs. From the perspective of RNA-binding proteins, both HuR and TIAR appear to modulate β-AR gene expression via their ability to interact with U-rich nucleotide sequences. From the perspective of microRNAs, it is well documented that they change in expression secondary to heart failure and increased β-AR signaling. In turn, several microRNAs are candidates to modulate β-AR expression, with miR let-7 being directly shown to do so. Previously, Wang and colleagues, demonstrated that overexpression of let-7 causes downregulation of the β-AR as well as an increased association of β 2 -AR mRNA with the Ago2/RISC complex. More recently, Liggett and colleagues have demonstrated that prolonged exposure to β-agonist drives a cAMP/PKA-dependent CREB-mediated increased let-7f expression, and in turn, downregulated β 2 -AR expression. Given the preservation of let-7 binding sites in the β 1 -AR 3′UTR, it would not be surprising if a similar regulatory pattern were seen.
Myopathic Potential of Individual Components of Adrenergic Receptor Pathways
The development and study of transgenic animal models has provided a wealth of information with regard to understanding the role of adrenergic receptor signaling in CHF. This section will summarize a few of the more relevant findings obtained from animal models containing cardiac-targeted overexpression or deletion of various components of adrenergic receptor pathways. The impact of these changes on the development or prevention of cardiomyopathy is discussed.
β 1 – and β 2 -Adrenergic Receptors
The idea that significant differences exist between β 1 – and β 2 -AR signaling in the heart has been reinforced by data from a number of transgenic mouse models. One of the more notable initial observations was that overexpression of each receptor subtype can result in strikingly different cardiac phenotypes. As originally reported by Milano and colleagues, overexpression of the wild-type β 2 -AR in mice, at relatively high abundance (∼50- to 200-fold), was associated with increased basal AC activity, enhanced contractility, and elevated left ventricle (LV) function. Interestingly, no pathology was apparent in animals up to 4 months of age. In a longer-term study, Liggett and colleagues reported comparable effects of increased β 2 -AR on the myocardium (i.e., enhanced cardiac function from birth); however, this group also noted linear gene dose-dependence with regard to the development of cardiomyopathy. Animals expressing more than 60× the background β-AR concentration maintained their hyperdynamic state for more than 1 year without an apparent increase in mortality. In contrast, overexpression of the β 2 -AR at ∼100-fold resulted in progressive cardiac enlargement, the development of heart failure, and premature death occurring at close to 1 year of age. At ∼350 times the level of wild-type expression, the β 2 -AR produced a cardiomyopathy, resulting in premature death in 50% of animals as early as week 25.
Compared to the extremely high levels of β 2 -AR expression required to produce myopathic results, wild-type β 1 -AR overexpression at relatively low abundance (∼20- to 40-fold) can lead to markedly decreased contractility and LV function and increased hypertrophy and fibrosis, resulting in progressive myocardial failure and premature death. The pathophysiological mechanism(s) responsible for the detrimental effects of β 1 -AR overexpression in these animals are only partially understood. Chronic increases in PKA activity are likely to be a component of the pathology associated with β 1 -AR overexpression, as overexpression of the catalytic subunit of PKA is, in its own right, cardiomyopathic. However, in the failing heart, cAMP abundance, and therefore PKA activity, is generally decreased. Thus, it is possible or perhaps even likely that β 1 -AR-mediated CaMKII activity, which is increased in CHF, underlies the observed pathology. Indeed, in a study by Dockstader and colleagues, where β 1 -AR Arg or Gly389 polymorphic variants were overexpressed, bioinformatic analysis detected a strong signature for the CaMKII pathway activation in Arg389 animals.
Initial studies reported an increase in systolic function in young animals overexpressing the β 1 -AR, with a progressive decline in LV function occurring with age. Interestingly, this appears to occur prior to any structural alterations, such as fibrosis, which do not become apparent until at least 4 months, depending on level of expression. In this same study, the mechanism of contractile dysfunction was found to involve impaired Ca 2+ handling in cardiac myocytes, characterized by marked prolongation of intracellular Ca 2+ transients. Subsequent examination of the expression levels of various SR proteins involved in Ca 2+ release and uptake revealed a modest increase in the expression of SERCA II protein in animals at 2 months of age, and increased phosphorylation of PLB at Ser16, a critical regulatory site, was also detected. Collectively, these results indicate that impaired Ca 2+ handling is likely another major causal factor in producing early contractile dysfunction in β 1 -AR overexpressors, with these changes occurring prior to the appearance of interstitial fibrosis or other structural alterations.
α 1 -Adrenergic Receptors
Both α 1a and α 1b -ARs are expressed at the protein level in human ventricular myocardium and neither one is downregulated in heart failure. Transgenic animal models that overexpress each subtype have been described. Overexpression of the wild-type α 1b -AR results in a dilated cardiomyopathy. In contrast, α1A transgenic cardiac overexpression produces an increase in contractility without hypertrophy. Importantly, double knockout of both α 1 subtypes results in the acceleration of remodeling in response to pressure overload, suggesting, on balance, that α 1 -ARs play an adaptive role in maintenance of myocardial chamber integrity. Moreover, of the two subtypes, the α 1a -AR appears to be particularly devoid of myopathic potential and is contractility enhancing, at least in rodents, the inference being that selective agonists may have therapeutic potential.
G-Proteins and Adenylate Cyclases
As described above, the G-proteins involved in adrenergic signaling are several, including Gs and Gi, which modulate AC activity, and Gq, which is linked to activation of PLC. Similar to that observed with the β-ARs, overexpression of each of these downstream signaling components results in a variety of cardiac phenotypes. Transgenic mice overexpressing wild-type Gαs have been described with this model, and are characterized by having a chronically increased heart rate, enhanced chronotropic and inotropic responses to agonist, altered β-AR density, and an increased frequency of cardiac arrhythmias. Over time, myocardial performance in Gαs-overexpressing animals declines and LV dilatation is observed. Likewise, myocyte hypertrophy, apoptosis, and fibrosis become evident with age, and animals die prematurely. In addition, there is evidence that overexpression of Gαs is associated with an increase in L-type Ca 2+ currents, an effect that appears to be independent of cAMP and AC activation. From these data, it has been suggested that Gαs may directly regulate the activity of L-type calcium channels. Interestingly, much like transgenic models of β-AR overexpression, the pathophysiological changes observed with Gαs overexpression can be prevented, and survival improved, through treatment with β-blockers.
Transgenic mice that overexpress Gαq also exhibit a myopathic phenotype characterized by marked myocyte hypertrophy, increased expression of hypertrophy-related genes, and increased fibrosis, ultimately resulting in decreased myocardial contractility. The mechanism of cardiomyopathy appears to involve, at least in part, cross-regulation to β-AR signaling pathways by Gαq or its downstream effectors. Mice that overexpress Gαq exhibit decreased AC activity in response to the agonist, which is apparently due to impaired functional coupling of β-ARs to AC, since β-AR density is unchanged when compared to wild-type expression levels. In addition, β-AR stimulation of L-type Ca 2+ channels is depressed in Gαq overexpressors, and expression levels of Gi are increased, as is PKC activity. In this model, the inhibition of Gαi unexpectedly caused sudden death, suggesting that the change in Gαi expression may be a compensatory mechanism to counteract other detrimental signaling caused by the Gq pathway.
Cardiac-targeted overexpression of a modified Gi-coupled receptor has been reported. Mice that contain this modified receptor reportedly exhibited significant ventricular conduction delays, which can be attenuated by treatment with pertussis toxin, strongly suggesting a Gi-dependent mechanism. Moreover, these animals developed a pronounced dilated cardiomyopathy, resulting in death by 15 weeks of age. These results were the first to suggest a potential causal role for increased Gi signaling in the development and progression of heart failure, and stand in distinction to the notion that the Gi pathway is simply protective, acting to abrogate the deleterious stimulatory effects of the Gαs pathway. In fact, overexpression of the carboxy-terminus of Gαi (GiCT), a peptide construct that inhibits Gi signaling, has been demonstrated to enhance apoptosis associated with ischemia.
In contrast to the above, overexpression of more distal components of the Gs signaling pathways, specifically ACs V or VI, does not appear to cause any form of cardiomyopathy. Agonist-stimulated AC activity is higher in this model, and basal and stimulated PKA activities are also increased; however, these effects translate into modestly enhanced inotropic, lusitropic, or chronotropic function. Further, no long-term histopathological sequelae or deleterious changes in cardiac function have been noted with AC overexpression. Based on this profile, preliminary (Phase 2) gene therapy trials utilizing adenovirus-mediated gene transfer of adenylyl cyclases have occurred. In this study, an adenovirus AC6 construct underwent intracoronary delivery in patients with an ejection fraction of less than 40%. It appears that AC6 delivery is both safe and efficacious in terms of increasing cardiac performance with larger follow-up trials likely being justified.
G-Protein Receptor Kinases
As described above, GRKs are central to modulating β-AR signaling and do so from a variety of perspectives. GRK-mediated phosphorylation of β-ARs desensitize and uncouple the receptors from their signaling pathways. They are the facilitators of receptor internalization and downregulation and, as mentioned earlier, in the case of GRK5, can affect cross-talk between canonical β-AR signaling pathways and other kinase pathways, including those of MAPKs and tyrosine kinases. Worth noting is the extensive work surrounding GRK2, which has been historically described as βARK2. Increased expression and/or activity of GRK2 has been implicated in progression of heart failure and post-myocardial infarction, and the use of a GRK2 mini-peptide that acts as an inhibitor appears to be of potential therapeutic benefit. The deleterious effects of GRK2 have been linked, in part, to PKA-mediated phosphorylation of the β 2 -AR leading to Gi-biased signaling, and to pro-death signaling via HSP90 mitochondrial targeting of GRK2.
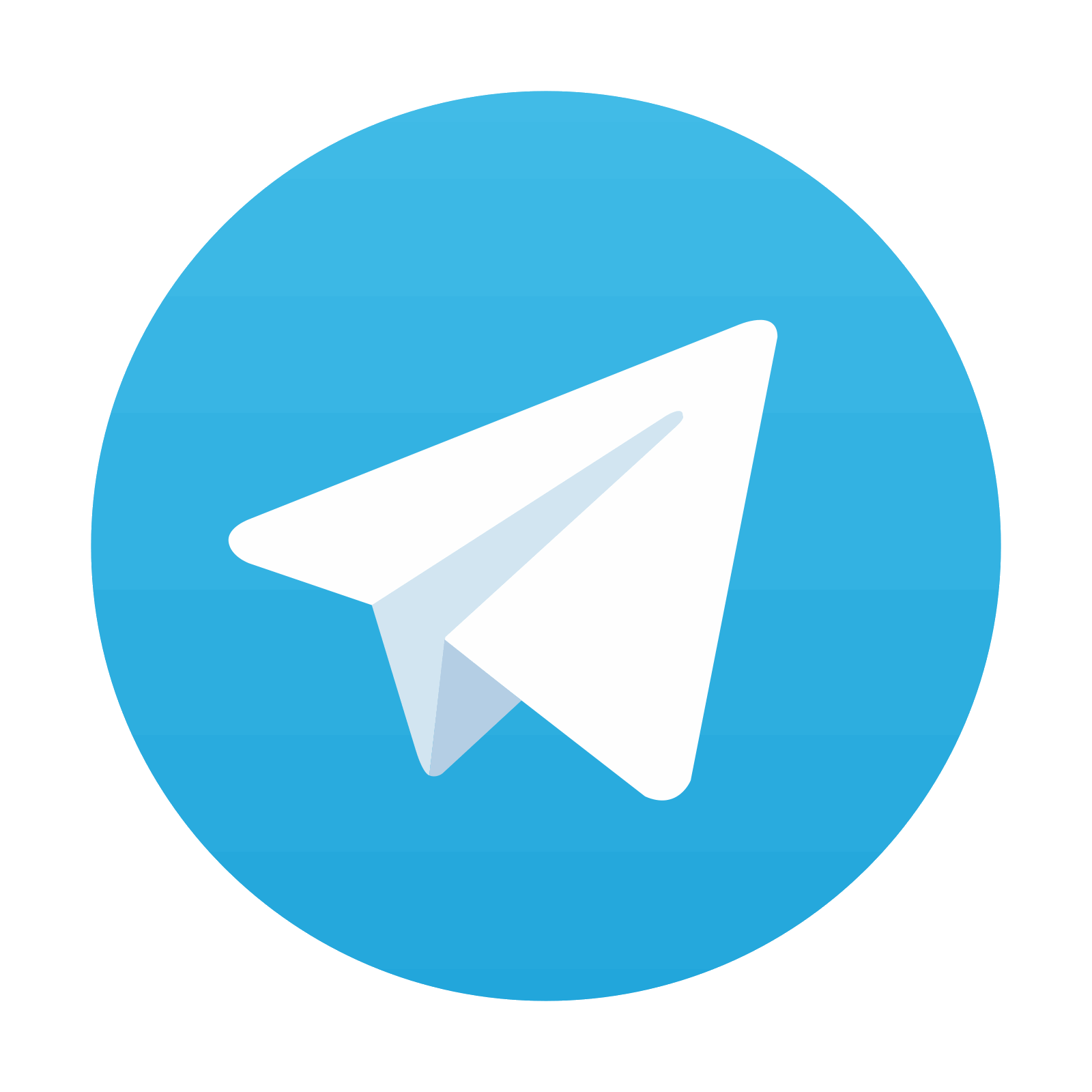
Stay updated, free articles. Join our Telegram channel
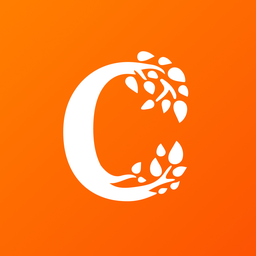
Full access? Get Clinical Tree
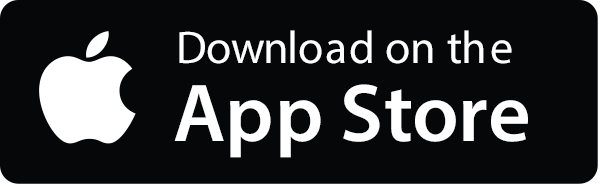
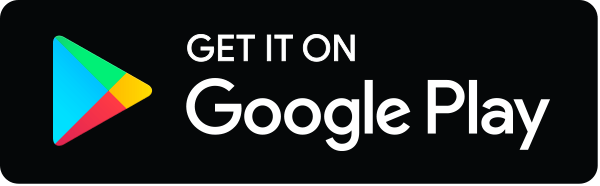