Active State, Length-Tension Relationship, and Cardiac Mechanics
T ension development and shortening by the heart depend on interactions between the contractile proteins (Chapter 4) and their interplay with the cytoskeleton and matrix proteins (Chapter 5). The classical studies of these mechanical properties described in Chapter 3 were carried out in tetanic contractions of frog sartorius muscle, whose parallel fibers are ideal for these analyses. Unlike the relatively simple mechanics of skeletal muscle, cardiac mechanics are complicated by the complex architecture of the heart, in which branched myocytes are organized in spiral bundles (Chapter 1). Furthermore, the heart cannot normally be tetanized, which makes it impossible to maintain the contractile element in a steady state. This poses a formidable obstacle because it requires that mechanical measurements be made under changing conditions, during the rise and fall of tension in each cardiac cycle. In spite of these difficulties in analyzing time-dependent and length-dependent changes in the contractile machinery, studies of the interplay between muscle chemistry and muscle performance provide valuable insights into the mechanical behavior of cardiac muscle.
Series Elasticity
Analyses of the interactions among the contractile proteins of living muscle are complicated by elasticities within the muscle. Efforts to understand the rise and fall of tension in skeletal muscle contractions led early investigators to postulate that a spring-like element, called the series elasticity, lies between the contractile element and the ends of the muscle (Fig. 6-1). Elongation of this elasticity at the onset of a contraction absorbs some of the energy generated when the contractile elements shorten. This contributes to a delay, called the latent period, between the time that a skeletal muscle is stimulated and the first appearance of tension.
When a skeletal muscle is stretched during the latent period, stiffness is found to have increased, which indicates that the contractile element had begun to stretch the series elasticity before tension appeared at the ends of the muscle. Absorption of energy by the series elasticity as tension develops at the onset of systole has important effects on the heart’s performance; conversely, dissipation of some of the tension in the series elasticity can be used to eject blood later during systole (see Chapter 12).
Twitch, Summation, and Tetanization
The brief response of a skeletal muscle to a single stimulus (S1 in Fig. 6-2) is a twitch. Because the skeletal muscle action potential is much briefer than the mechanical response, these muscles can respond to a second stimulus before they begin to relax (S2 in Fig. 6-2). The tension developed in the second contraction is greater than that developed during the first, which is why this phenomenon is called summation. If a skeletal muscle is stimulated so rapidly that
each successive stimulus arrives before the muscle has begun to relax, tension continues to rise until it reaches a new steady state (Fig. 6-3). The strong sustained contraction is called a tetanus, and the new level of tension is called tetanic tension. In a skeletal muscle contracting under isometric conditions, tetanic tension exceeds twitch tension approximately threefold and corresponds to P0 of the force-velocity curve described in Chapter 3. Twitch tension is less than tetanic tension because energy absorbed by stretching of the series elasticity reduces the amount of the force developed by the contractile element that is transmitted to the ends of the muscle (see below).
each successive stimulus arrives before the muscle has begun to relax, tension continues to rise until it reaches a new steady state (Fig. 6-3). The strong sustained contraction is called a tetanus, and the new level of tension is called tetanic tension. In a skeletal muscle contracting under isometric conditions, tetanic tension exceeds twitch tension approximately threefold and corresponds to P0 of the force-velocity curve described in Chapter 3. Twitch tension is less than tetanic tension because energy absorbed by stretching of the series elasticity reduces the amount of the force developed by the contractile element that is transmitted to the ends of the muscle (see below).
![]() Fig. 6-1: Model of muscle showing the contractile element in series with a spring-like series elasticity. |
Tetanic contraction, tetanus, and tetany are not the same. Although the term “tetanus” is used to describe both a tetanic contraction and the disease caused by the endotoxin of the bacteria Clostridium tetani, the distinction is obvious. Tetany, which differs from both, is a hyperexcitable state of skeletal muscle caused when lowering of the threshold of the motor endplate to physical and chemical stimuli allows contractions to appear in response to what are normally subthreshold stimuli. This pathological condition can be caused by systemic alkalosis or hypocalcemia.
The Series Elastic Element
Figure 6-1 follows a tradition that shows the series elastic element as separate from the contractile element. In skeletal muscle, some of this elasticity is in the tendinous ends of the muscle. Stretching of damaged regions adjacent to the clamps used to hold isolated muscles add to this
elasticity, as does asynchrony of contraction caused by asynchronous stimulation. Significant elasticity is also found in the sarcomeres, much of which is attributable to elasticities in the cross-bridges, titin, and other components of the cytoskeleton.
elasticity, as does asynchrony of contraction caused by asynchronous stimulation. Significant elasticity is also found in the sarcomeres, much of which is attributable to elasticities in the cross-bridges, titin, and other components of the cytoskeleton.
![]() Fig. 6-3: A twitch, as shown in Figure 6-2 (left), and a tetanic contraction (right) in a skeletal muscle contracting under isometric conditions: Application of a train of stimuli (S) generates a series of responses that cause tension to rise to a much higher level in a tetanus than is developed during a twitch. Tetanic tension remains at this high level until stimulation ends or the muscle fatigues. |
Active State in Skeletal Muscle
The tension recorded at the ends of a muscle during a twitch is less than that generated by the contractile element because the series elasticity absorbs some of the mechanical energy released by the contractile proteins. The influence of this elasticity can be eliminated by applying a “quick stretch” or a “quick release” to the ends of the muscle. In skeletal muscle, these experiments reveal the remarkably rapid development of an active state, which is the tension developed by the interactions between the myosin cross-bridges and actin. In cardiac muscle, however, similar studies yield results that are quite different from those in skeletal muscle (see below).
Quick-Stretch Experiments
One way to compensate for the absorption of mechanical energy by the series elasticity during the onset of a twitch is to stretch the muscle very rapidly. The key to understanding quick-stretch experiments is to realize that the rapid increase in muscle length can, if the stretch is “just right,” equalize tension at three places: between the ends of the muscle, within the contractile element, and across the series elasticity. When this occurs, the tension recorded at the ends of the muscle will be the same as the active state tension developed by the contractile element.
![]() Fig. 6-4: Measurement of the active state by application of quick stretches to a skeletal muscle contracting under isometric conditions. Solid line: Twitch, as shown in Figures 6-2 and 6-3. Dashed lines: 1: When the muscle is quickly stretched a relatively short distance immediately after stimulation, the tension at the end of the stretch is less than that produced by the contractile element; as a result, tension continues to increase. 2: When the muscle is quickly stretched a relatively long distance immediately after stimulation, the tension at the end of the stretch exceeds that developed by the contractile element; as a result, tension declines after the stretch. 3: When the muscle is quickly stretched to a length at which tension is the same as the active state of the contractile element, tension remains at a plateau that equals the active state. |
In quick-stretch experiments, the tension developed by the muscle depends on the extent to which its length is increased. This is depicted in Figure 6-4, which shows the tension recorded after a skeletal muscle is stretched to three different lengths immediately after stimulation. If the stretch is small, tension will be less than that which can be generated by the interactions between the myosin cross-bridges and actin; as a result, the contractile element will develop more tension (curve 1 in Fig. 6-4). If, on the other hand, the muscle is stretched to a length so long that the tension across the muscle exceeds that developed by the contractile element, the latter will lengthen, causing tension to decrease until it equals the tension developed by the contractile proteins (curve 2 in Fig. 6-4). If the quick stretch is “just right,” and brings the tension between the ends of the muscle to the same level as that developed by the contractile element, the muscle will neither lengthen nor shorten; instead, tension will remain at a level equal to that of the contractile element (curve 3 in Fig. 6-4). This tension is the active state.
The time course of active state development can be estimated by applying quick stretches at different times after stimulation (Fig. 6-5). When such experiments are done with skeletal muscle, active state is found to develop very rapidly, reaching a brief plateau before twitch tension reaches its peak, after which the active state begins to decline.
Because muscle tension exceeds active state tension at the end of a twitch (Fig. 6-5, inset), energy stored in the stretched series elasticity is returned to the ends of the muscle; this allows some of the energy that had been expended to stretch the series elasticity to be used to perform external work. Use of energy stored in the stretched series elasticity is especially important in the heart, where the balance between energy supply and energy demand is precarious even under normal conditions (see Chapter 2). One reason that allowing a ventricle to eject increases its efficiency (see Chapter 12) is that the decrease in wall stress which normally occurs during ejection allows some of this elastic energy to be used to pump blood, rather than being degraded to heat.
The damping effect of the series elasticity explains why more tension is developed during a tetanus than during a twitch. In the latter, the tension between the ends of the muscle decreases when the series elasticity is stretched, whereas during a tetanus, repeated stimulation prevents the active state from declining, which allows active state tension to become the same as the tension
at the ends of the muscle (Fig. 6-6). Tetanic tension therefore measures the tension developed by the contractile element.
at the ends of the muscle (Fig. 6-6). Tetanic tension therefore measures the tension developed by the contractile element.
Quick-Release Experiments
The ability of a muscle to develop tension and shorten reflects two different properties of the contractile process (see Chapter 3). One is the number of rigor bonds, which determines both active state tension and P0, the maximum tension that a tetanized muscle is able to generate. The other property of contracting muscle, the velocity of contractile element shortening (Vmax),
is an index of the rate of cross-bridge turnover, which is independent of the number of rigor bonds. The latter can be evaluated by subjecting the contracting muscle to “quick releases.”
is an index of the rate of cross-bridge turnover, which is independent of the number of rigor bonds. The latter can be evaluated by subjecting the contracting muscle to “quick releases.”
When a skeletal muscle that has been tetanized under isometric conditions is suddenly presented with a series of new, reduced loads, the shortening that follows the quick releases occurs in two phases (Fig. 6-7). The first is a very rapid decrease in length that is caused by shortening of the series elasticity (dashed line in Fig. 6-7). The velocity of the second slower phase of shortening, which is the result of shortening of the contractile element (dotted lines in Fig. 6-7), increases when the quick releases are made to progressively lighter loads (1, 2, and 3 in Fig. 6-7, going from heaviest to lightest). Plots of the load dependence of shortening velocity in this second phase yield hyperbolic curves (Fig. 6-8) that are similar to the force-velocity relationship described in Chapter 3 (Fig. 3-15). Quick-release experiments in skeletal muscle can therefore be used to measure Vmax, an index of the rate of cross-bridge cycling, as well as P0, an index of the number of active force-generating sites. In cardiac muscle, however, the results of quick-release experiments are very different from those shown in Figure 6-7.
The Length-Tension Relationship
A major determinant of the ability of a skeletal or cardiac muscle to develop tension is its resting length. Curves describing this length-tension relationship (Fig. 6-9) are customarily scanned from left to right, so that the increase in developed tension that occurs when a muscle
is stretched at shorter muscle lengths is called the ascending limb, while the decline of tension when the muscle is stretched at longer lengths is the descending limb. The tension developed during tetanic contractions in skeletal muscle is maximal at intermediate muscle lengths (lmax or l0).
is stretched at shorter muscle lengths is called the ascending limb, while the decline of tension when the muscle is stretched at longer lengths is the descending limb. The tension developed during tetanic contractions in skeletal muscle is maximal at intermediate muscle lengths (lmax or l0).
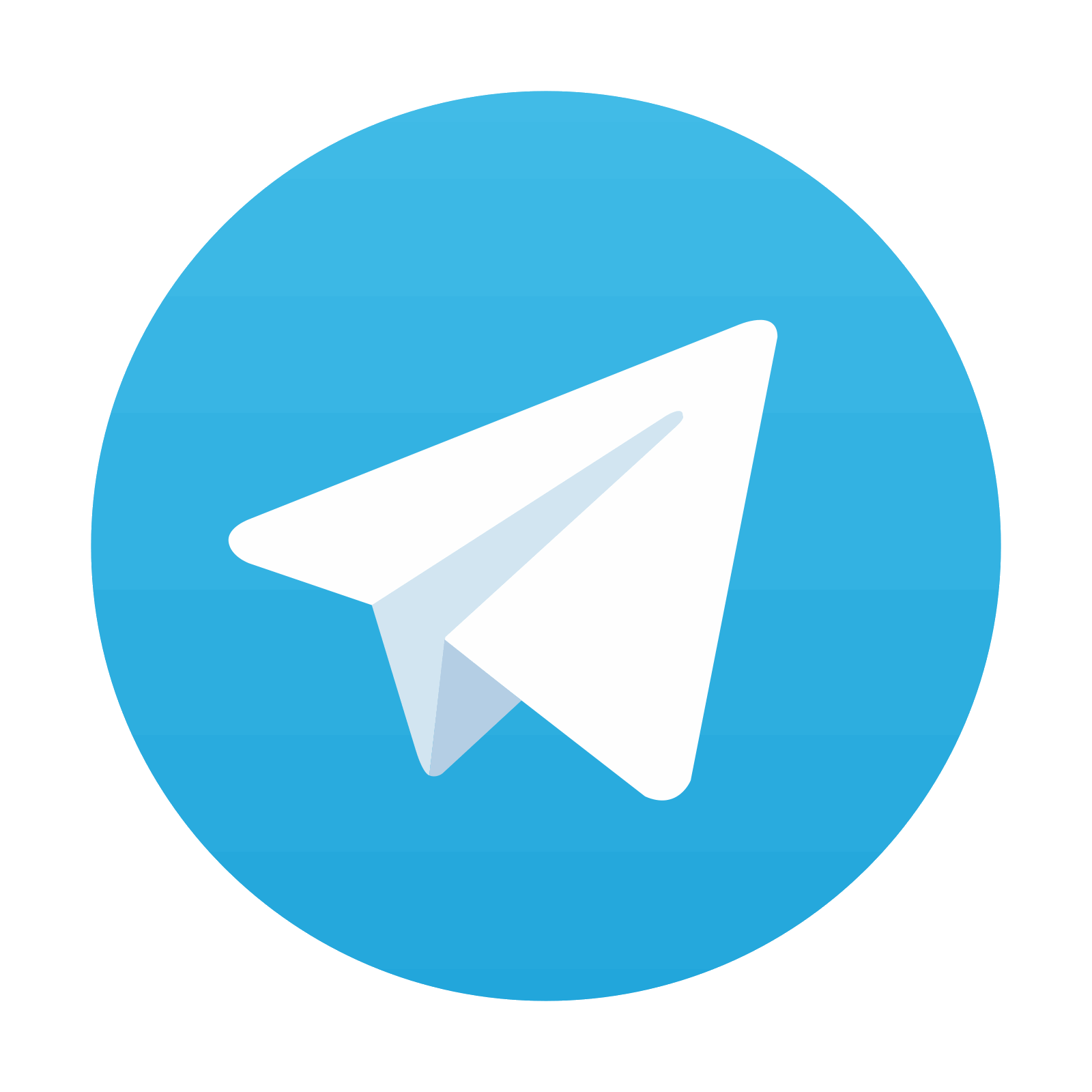
Stay updated, free articles. Join our Telegram channel
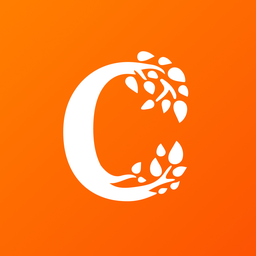
Full access? Get Clinical Tree
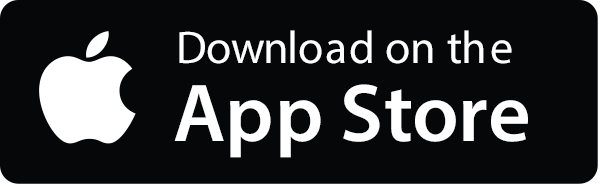
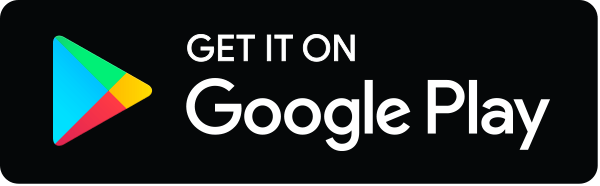