Abstract
Ventricular tachycardia (VT) can develop late after myocardial infarction, often in the context of advanced systolic dysfunction, potentially resulting in hemodynamic instability and sudden death. Although implantable cardioverter-defibrillators are generally required to address this risk, multiple shocks to treat VT are associated with significant morbidity and mortality. Catheter ablation is an important option for the treatment of postinfarct VT, particularly in view of the low efficacy and potential toxicity of antiarrhythmic drug therapy. The most common arrhythmogenic mechanism in these patients is reentrant excitation through the infarct scar with the surface 12-lead electrocardiogram providing a useful guide to the region of wave front exit from the scar border. Mappable VT circuits can be defined using the response to overdrive pacing (entrainment), but this can only be applied in the minority of VTs. Substrate mapping techniques have evolved considerably since the original description. In addition to bipolar signal voltage, multiple additional electrogram parameters and pace mapping responses are used to define the footprints of potential VT circuits in sinus rhythm, often with multipolar catheters. Following a detailed characterization of the substrate, an ablation strategy that incorporates most of the critical elements into the lesion set is delivered. VT noninducibility remains the most commonly used procedural end point for postinfarct VT but has significant limitations. The results of ablation have improved in the current era and recent randomized trials show prognostic benefit over antiarrhythmic drug therapy with acceptable complication rates. This chapter will examine the mechanistic basis of postinfarct VT and the evolution of catheter ablation as a successful treatment for it.
Keywords
catheter ablation, entrainment, myocardial infarction, substrate mapping, ventricular tachycardia
Key Points
Mechanism
- •
The mechanism of postinfarction monomorphic ventricular tachycardia (VT) is generally reentrant myocardial excitation through surviving myocyte bundles within the infarct scar.
Diagnosis and Mapping
- •
The diagnosis is made by excluding supraventricular and preexcited causes of broad complex tachycardia. Activation, entrainment, and substrate mapping techniques may all required to localize critical VT circuit components.
Ablation Targets
- •
The basic critical target for ablation of postinfarct VT is the narrow diastolic isthmus constrained by anatomic dense scar regions as well as by functional barriers. Presystolic and mid-diastolic electrograms are recorded from isthmus sites during tachycardia, and their involvement in VT can be proven by entrainment mapping. For nonmappable VT, sinus rhythm correlates of isthmus sites need to be defined by electrogram analysis and pacing. This process is called substrate mapping and localizes the infarct scar and border zone, sites of slow conduction with good pace maps, and sites of late-activating isolated potentials.
Special Equipment
- •
Electroanatomic mapping systems are required for defining substrate, tagging critical target sites, and recording ablation lesion delivery. Preoperative scar imaging and integration into the mapping system may be helpful. Multipolar catheters with narrow-spaced bipoles and ultrahigh-density mapping have improved visualization of VT circuits and the underlying substrate. Second-generation open-irrigation ablation catheters and contact force sensing have improved the biophysics of lesion delivery and have the potential to allow safer creation of larger lesions with lower thromboembolic risk.
Sources of Difficulty
- •
Hemodynamic instability and inability to map VT in patients with advanced structural heart disease make these procedures challenging. Multiple inducible VT morphologies are the rule. Effective lesion delivery within dense scar may be difficult with currently available energy sources.
Introduction
The development of sustained monomorphic ventricular tachycardia (VT) is one of the most significant late complications that can occur in a patient who has suffered a prior myocardial infarction (MI). Regardless of the hemodynamic tolerability of any index episode of VT in this setting, patients with a healed MI scar and significant left ventricular (LV) systolic dysfunction have substantially reduced survival and derive a demonstrable prognostic benefit from implantable cardioverter-defibrillator (ICD) placement, even before developing clinical VT. It is clear, however, that ICDs are deployed for sudden death risk mitigation and should not be considered a treatment or cure for VT. Repeated ICD shocks for recurrent VT can lead to significant morbidity and mortality, and repeated delivery of antitachycardia pacing may also be associated with adverse outcomes. Present-day medical therapy is incompletely effective in preventing ICD therapy and comes with the risk of significant adverse effects and additional extracardiac toxicity in the case of amiodarone. Few meaningful breakthroughs in antiarrhythmic drug development have occurred in recent times to suggest any change in this landscape is imminent.
In this light, catheter ablation assumes an important role in the overall management of ischemic cardiomyopathy (ICM) patients with VT. Although the suggestion of a prognostic benefit has been found in one trial, and although the prevention of ICD shocks may lead to a secondary survival benefit, the primary goal of the procedure is to improve quality of life by prevention of VT recurrences and ICD therapies without antiarrhythmic drug side effects.
Anatomy
Multiple intracardiac mapping studies have confirmed that the electrophysiologic mechanism of the vast majority of monomorphic VT in the setting of prior MI is reentry. As clearly demonstrated in the early seminal surgical studies, and confirmed subsequently by entrainment mapping data, critical portions of the reentry circuitry of postinfarct VT reside within a healed myocardial infarct scar, usually a large and confluent one ( Fig. 30.1 ). Such scars are often associated with advanced LV remodeling, areas of dyskinesis and aneurysm formation, and significant LV systolic dysfunction. Infarct scar formation and remodeling is not a uniform amorphous process; instead structural and ultrastructural heterogeneity within scar regions is universal ( Fig. 30.2 ). Collateral formation, endoluminal perfusion, acute reperfusion therapy, and ongoing collagen turnover all contribute to the formation of surviving (predominantly subendocardial) myocyte bundles of varying diameter interlaced in networks through the dense infarct core. These bundles are separated by insulating sheets of collagen that alter wave front propagation through scar in a manner conducive to the establishment of reentrant tachyarrhythmias. The extent of ventricular scar and LV systolic dysfunction in patients with ICM is an important determinant of arrhythmic risk. Sustained monomorphic VT is more likely seen in those with larger infarct scars. The advent of primary prevention medical therapy and earlier, more effective acute reperfusion therapy with primary percutaneous intervention have altered infarct scar characteristics. Patchy fibrosis is now seen more frequently, and aneurysm formation has become rare. This has altered the frequency and characteristics of VT seen in the chronic phase after MI with more rapid VT seen in such patients. It remains unclear whether the reduced late VT incidence expected with smaller healed infarcts will be balanced by an increased prevalence of VT as long-term post-MI survival improves.


Remember that not all patients with reentrant VT and coronary artery disease have a myocardial infarct-related ventricular scar and some may instead have a coexisting nonischemic dilated cardiomyopathy. Such patients tend to have basal VT sources as well as the frequent epicardial and intramural VT exits typical of that substrate, in direct contrast to postinfarct ICM patients whose pathology and VT circuitry characteristically lie in the subendocardial layer.
Pathophysiology
Disorders of repolarization or disorders of conduction (or both) may form the basis of the electrophysiologic substrate in any arrhythmogenic disease, but in the postinfarct context, abnormalities of conduction predominate. Myocardial necrosis at the time of the initial infarction and replacement of lost myocytes with collagen-based fibrous tissue leads to an overall reduction in the number and density of gap junctions between surviving cells within an infarct scar. Thus even though they are capable of generating recordable action potentials, these myocytes are poorly coupled electrically to nearby cells. Not only does this lead to a slowing of wave front conduction velocity in the scar, but also, because of source-sink mismatch, a greatly increased likelihood of conduction failure producing unidirectional block. In addition, electrical propagation is significantly more affected in the transverse direction orthogonal to myofiber axis as a result of an amplification of normal nonuniform anisotropy by layers of collagen between myocyte bundles. This slow, discontinuous wave front conduction within an infarct scar sets up the ideal milieu for the establishment of reentrant excitation, with the constrained diastolic isthmus usually located within the core of the scar. The exit of the VT wave front from this isthmus occurs at the scar border zone where inscription of the surface QRS commences. The wave front then propagates through the ventricle surrounding the scar, often in a figure-of-8 (dual loop) configuration, before reentering the protected isthmus within the scar again. The diastolic corridor may in some cases be constrained between dense infarct scar and an anatomic barrier such as a valve annulus or a surgical conduit. The contribution of functional barriers to the complete VT reentrant circuit have been underestimated but older resetting studies and recent ultrahigh resolution activation mapping studies confirm their importance, particularly at the entrance to and exit from the diastolic corridor. Approximately 40% of VTs have isthmuses that are shared with a second VT. Evidence that supports reentry as the dominant mechanism of postinfarct VT is summarized in Box 30.1 with some examples in Fig. 30.3 .
Induction and termination with programmed ventricular stimulation
Site-specificity of induction
Direct relationship between coupling interval of the initiating extrastimulus and the onset of the first beat of VT
Continuous electrical activity related to VT onset and perpetuation recorded by an electrode near the slow zone
Recording of mid-diastolic potentials that cannot be dissociated from VT
Extrastimulus-mediated resetting of VT with fusion
Entrainment of VT with overdrive pacing
Electroanatomic activation map of the ventricle containing >90% of the VT cycle length
Termination of VT with global noncapture from a nonpropagated extrastimulus
VT , Ventricular tachycardia.


The anatomic substrate in the postinfarct patient can be inferred from the presence of Q waves on the surface electrocardiogram (ECG), demonstrated by scar imaging techniques such as late gadolinium enhancement (LGE) on magnetic resonance imaging (MRI) or even visualized directly during surgical subendocardial resection. However, the electrophysiologic substrate can really only be defined by the use of invasive catheter-based electrode recordings at an electrophysiology study (EPS).
At EPS, the induction of sustained monomorphic VT with the use of programmed stimulation implies that the electrophysiologic substrate for VT exists although this does not necessarily predict the presence of spontaneous clinical VT because specific triggers such as ventricular ectopy, heart failure, or changes in autonomic tone are required for this to occur. In addition, at EPS, catheter-based electrode recordings from the infarct region show characteristic abnormalities reflective of the loss of functioning cardiomyocytes and the slow and discontinuous wave front conduction present in ventricular scar. Normal ventricular myocardial bipolar electrograms are sharp, biphasic or triphasic signals of greater than 1.5 mV peak-to-peak amplitude, 70 ms or less duration, and/or greater than 0.046 amplitude:duration ratio ( Fig. 30.4 ). A loss of cardiomyocyte mass in the scar results in attenuation of the bipolar electrogram amplitude with dense scar showing bipolar voltage less than 0.5 mV and regions of bipolar voltage between 0.5 and 1.5 mV typically being seen in the scar border. Slow conduction in the infarct scar causes prolongation of the local bipolar electrogram with multiple low-amplitude deflections ( Fig. 30.5 ). Such electrograms are called fractionated and are defined by a bipolar voltage less than 0.5 mV, a duration of more than 133 ms, and/or an amplitude:duration ratio of less than 0.005 29 (see Fig. 30.4 ). The multiple deflections of fractionated electrograms have been shown to each correspond to delayed activation of surviving islands of myocyte separated by dense collagen sheets within the field of view of the recording electrode. When activation of a discrete surviving myocyte bundle is sufficiently delayed, a low-amplitude isolated late potential (ILP) may be recorded following an isoelectric interval after the larger initial, usually far-field, ventricular electrogram ( Figs. 30.4 and 30.5 ). Such a bundle may form an anatomically constrained conducting channel in the scar that has the potential to serve as the protected diastolic isthmus of a VT circuit (see Fig. 30.5 ).


In the setting of coronary disease, polymorphic VT may suggest the presence of acute ischemia. There may be multiple operative mechanisms, including abnormal automaticity or Purkinje fiber mediated reentry or triggered activity from diastolic calcium overload in compromised but still viable cells. Polymorphic VT does not require the presence of a healed anatomic infarct substrate as it can occur in patients with no prior ventricular scar presenting with acute coronary syndromes for the first time. It may occur in the very early phase of infarct repair and remodeling where Purkinje fiber triggers at the border of the necrotic zone may be important in its initiation ( Fig. 30.6 ).

Arrhythmia Diagnosis and Differential Diagnosis
The vast majority of patients with sustained postinfarct VT can be diagnosed by a 12-lead ECG recorded at the bedside during tachycardia. It is almost axiomatic that a broad complex tachycardia in the setting of a patient with a prior MI is VT, but multiple published algorithms have been suggested to help exclude the other less likely differential diagnoses, namely supraventricular tachycardia with aberrant conduction (including atrial flutter with 1:1 conduction), preexcited tachycardia, paced tachycardias, and artifact ( Fig. 30.7 ).

The diagnostic criteria for postinfarct scar-related VT are listed in Box 30.2 . The diagnosis of myocardial scar-related VT hinges on the demonstration of atrial, atrioventricular nodal, and His-Purkinje dissociability from the tachycardia (which excludes all forms of supraventricular, preexcited, and fascicular tachycardias, including bundle branch reentry VT). Such proof can be obtained at the bedside with the surface 12-lead ECG or in the laboratory during EPS with pacing maneuvers. In the presence of a dual-chamber ICD system, the presence of ventriculoatrial dissociation on ICD interrogation is essentially conclusive. The diagnosis of VT can also be strongly suggested in patients with single-chamber ICDs as stored electrogram morphology in tachycardia should be different from the sinus rhythm conducted morphology in VT. However, right bundle branch block aberrance during supraventricular tachycardia can alter the wave front vector by which the sensing bipole in the right ventricle (RV) is activated and hence also changes the ICD electrogram morphology. Such ICD intracardiac electrograms may be useful in matching induced VTs to clinical arrhythmias as well as potentially even in pace mapping ( Fig. 30.8 ). The latter maneuver may be the only mapping option in the uncommon scenario in which no VT is inducible at EPS in a patient in whom there are no 12-lead ECG recordings of clinical VT.
Broad complex tachycardia with demonstrable atrial and AV nodal dissociation
Surface QRS morphology inconsistent with SVT or preexcited tachycardia (e.g., negative precordial concordance, QS complex in V6)
Surface QRS width in VT narrower than conducted basal rhythm QRS complex
Sinus rhythm HV interval longer than during tachycardia (except bundle branch reentry)
Dissociation of His-Purkinje activation from VT (except bundle branch or interfascicular reentry)
V-V interval precedes and predicts H-H interval during cycle length wobble
Atrial overdrive pacing changes or narrows QRS complex and/or results in an A-V-V-A response if tachycardia continues after cessation of pacing
AV , Atrioventricular; SVT , supraventricular tachycardia; VT , ventricular tachycardia.

Periprocedural Considerations
Until recently, catheter ablation for postinfarct VT has generally been used as a near-last resort treatment option, typically reserved for the patient with multiple ICD shocks refractory to repeated device reprogramming who has exhausted all antiarrhythmic drug options, including high-dose amiodarone. With improvements in mapping and ablation, the procedure has increasingly been offered at an earlier stage in the natural history, and some data suggests that this may increase the efficacy of postinfarct VT ablation. Two large randomized trials have shown that upfront prophylactic catheter ablation before or after secondary prevention ICD insertion reduces the incidence of subsequent arrhythmia events. The recent Ventricular Tachycardia Ablation versus Escalation of Antiarrhythmic Drugs (VANISH) study of ICM patients with recurrent VT despite antiarrhythmic drug use showed a significant benefit of catheter ablation over escalation of drug therapy in reducing the composite primary outcome measure of death, VT storm, and appropriate ICD shock. However, catheter ablation in the context of these often very sick ICM patients can be challenging and hazardous, with major complication rates of up to 8% described. Thus careful preoperative patient assessment and meticulous periprocedural risk management are vital to optimize safety and efficacy.
Preprocedural planning begins with a careful review of all clinical arrhythmia data from 12-lead ECGs, telemetry strips, and stored ICD electrograms. The number and likely exits of all clinical VTs are determined. The patient’s clinical condition is assessed with respect to their ability to tolerate a prolonged procedure, often with multiple VT inductions and significant intravascular fluid loading. Heart failure decompensation and myocardial ischemia in particular must both be reversed as much as possible before commencing ablation. The presence of peripheral arterial disease is an important comorbidity from the viewpoint of both retrograde transaortic vascular access and potential hemodynamic support options such as an intraaortic balloon pump (IABP). The number and status of all coronary bypass grafts and stents should be ascertained and myocardial revascularization completed as appropriate. Prior cardiac surgery will generally preclude unrestricted percutaneous pericardial access for catheter ablation (because of the presence of dense adhesions or bypass grafts), but this is necessary in postinfarct VT patients given the predominantly subendocardial location of their arrhythmogenic substrate. Prior valvular surgery is an important consideration, with mechanical prosthetic valves in the aortic and mitral positions precluding retrograde and transseptal access to the LV respectively. The rare situation of dual mechanical prosthetic valves may require transapical or interventricular septal access to the so called “no-entry ventricle.”
Oral anticoagulation reversal needs to be carefully considered before scar-related VT ablation because even a planned exclusively transseptal approach may require additional retrograde or epicardial access and/or IABP placement.
A preoperative echocardiogram is performed to quantify LV size and systolic function as well as to assess for the presence of intracardiac thrombus. It should be noted that the latter is not an absolute contraindication to an endocardial ablation attempt, particularly with laminated chronic thrombi, although these may hinder catheter access to arrhythmogenic subendocardial regions.
Conscious sedation may be preferred for this procedure over general anesthesia wherever possible because of improved hemodynamics, both in VT and sinus rhythm, as well as easier VT induction. However, patient tolerance is an important consideration in these often protracted and difficult procedures, and administration of conscious sedation by specialist anesthetic staff allows for close patient monitoring, precise adjustment of sedation depth, and early general anesthetic induction if required. A urinary catheter is vital to monitor fluid balance adequately in the face of large fluid loads from open-irrigation catheters as well as to warm of reduced peripheral and splanchnic perfusion and impending cardiogenic shock. In patients with very dilated, poorly contractile ventricles, consideration should be given to upfront hemodynamic support with IABP or percutaneous LV assist device.
A detailed assessment of the anatomic substrate is important to properly plan the procedure and anticipate potential challenges. This begins with the 12-lead ECG that may provide useful information on infarct location both in sinus rhythm as well as in VT. Regional wall motion abnormalities on echocardiography, with or without the use of acoustic contrast or speckle tracking strain imaging, may define the infarct scar satisfactorily. However, the current gold standard for scar imaging is LGE on MRI. The superb soft tissue contrast and resolution provided by this imaging modality can provide a detailed assessment of scar architecture and can be used for image integration with the electroanatomic mapping system. This may help to identify likely VT isthmus locations and improve the efficiency of the procedure. Patients with ICDs can be scanned at experienced centers with the appropriate safety precautions. Susceptibility artifact from the pulse generator and leads remains an issue although newer MRI technologies such as wideband LGE may reduce this also. Patients who cannot undergo MRI scanning may have scar imaging performed with positron emission tomography (PET) and/or multidetector computed tomography (CT) to obtain a high resolution metabolic scar map that can also be used for image integration.
Intraprocedural imaging is an important consideration in VT ablation, where it is needed for defining substrate, tagging ablation targets, tracking lesion location, and monitoring for complications. Whereas fluoroscopy and electroanatomic mapping are deployed universally because of widespread availability and ease of use, intracardiac echocardiography (ICE) has been used increasingly for VT ablation procedures. It allows for real-time scar imaging and visualization of catheter contact and lesion formation in addition to assisting with percutaneous pericardial access if this is required. Another benefit of ICE is its unique ability to provide real-time monitoring for complications such as stream pop, thrombus formation, and cardiac perforation. This is particularly useful in VT ablation as hypotension frequently occurs during these procedures and ICE can rapidly exclude tamponade as a cause of this. In addition, ICE is the main online imaging modality that allows visualization and ablation of endocavitary structures such as the papillary muscles, which can be involved in inferior MI scars. The future of intraprocedural imaging in VT ablation is likely to reside with interventional MRI technologies, which will allow for definitive online substrate mapping and gold-standard assessment of lesion formation with thermography.
Mapping Strategies
Fundamentally, the aim of mapping VT (or any arrhythmia) is to define the electrophysiologic mechanism and to localize the critical arrhythmogenic components that may be targeted by catheter ablation. For focal VTs, the critical arrhythmogenic site is the point source of origin, and this can be localized with precision using activation mapping and pace mapping alone. In activation mapping, the goal is to localize the site with the earliest presystolic activation time before the QRS is inscribed. Pace mapping relies on the principle that pacing at the site of origin of a tachycardia should theoretically recapitulate the QRS morphology perfectly. Both of these techniques are ideally suited to mapping the site of origin of focal VTs, the majority of which occur in the absence of structural heart disease. In the postinfarct context, however, the vast majority of VT is caused by macroreentry through the infarct scar and, as with any reentrant circuit, terms such as “site of origin” and “earliest” are meaningless. The goal of mapping reentrant VT is to define the narrowest part of the reentrant circuit (the diastolic isthmus) where it is most susceptible to interruption with ablation lesions. In reentry, activation mapping can be used to define the boundaries and direction of rotation of the circuit, with progressively earlier signals mapped further proximally in the protected diastolic corridor. The use of the 3-dimensional electroanatomic systems aids in visualization of such activation maps. However, multiple technical limitations prevent routine activation mapping of scar-related reentrant VT, and for this reason, postinfarct VT is mostly mapped by its response to overdrive pacing (entrainment mapping).
Once vascular access has been obtained and diagnostic catheters have been placed in the heart, VT is induced with programmed stimulation or by catheter manipulation during electroanatomic map creation. Induced VTs are analyzed for cycle length and/or morphology match to clinical VTs and are assessed as to whether they are mappable by conventional activation and entrainment mapping. In addition to hemodynamic tolerability, the other criteria required to make VT mappable are listed in Box 30.3 . Mappable VTs comprise the minority of VTs induced in ICM patients, although most patients have at least one mappable VT morphology. With mappable VTs, ascertainment of circuit components and ablation of critical targets occurs during tachycardia. This allows for assessment of the response to radiofrequency energy delivery, with immediate or early VT termination during lesion delivery being an encouraging sign of elimination of that VT.
VT is reproducibly inducible
VT is sustained
VT is monomorphic
VT cycle length is regular
VT is hemodynamically tolerated
VT does not transition to other morphologies
VT does not terminate or change morphology/rate with overdrive pacing
VT , Ventricular tachycardia.
Unmappable VTs are those that do not allow for entrainment mapping to be performed, often but not exclusively caused by hemodynamic embarrassment. Strategies that may help make nontolerated VTs mappable include the use of inotropes or mechanical circulatory support with IABP or percutaneous left ventricular assist device. Substrate mapping and noncontact mapping approaches can be used for genuinely unmappable VTs and are considered further subsequently. In general, a combination of mapping strategies is needed at most scar-related VT ablation procedures.
Activation Mapping
Using a meticulous point-by-point approach, de Chillou et al. performed 3-dimensional contact activation mapping of 33 tolerated VTs in 21 patients. Either single or dual loop macroreentrant circuits were demonstrated as the mechanism for all VTs, with protected diastolic isthmuses bounded by parallel conduction barriers such as myocardial scar or valve annuli. Various fractionated and mid-diastolic electrograms were recorded from such regions with a 3.5-mm-tip ablation catheter. Isthmus dimensions measured 18 to 41 mm in length and 6 to 36 mm in width and linear ablation across these isthmuses treated VT effectively, with a low 10% recurrence rate at 16 ± 8 months follow-up. Although this important study contributed to the mechanistic understanding of postinfarct VT, until recently, routine point-by-point activation mapping of the entire VT circuit was impractical, time-consuming, unnecessary and, in isolation, usually unhelpful to precisely define the narrowest portion of the protected diastolic pathway. Annotation of long duration, multicomponent fractionated and double potentials during activation mapping was particularly difficult, and mid-diastolic potentials (MDPs) may not have represented critical isthmuses but rather passively activated bystander cul-de-sacs or outer loops.
However, the advent of an ultrahigh resolution electroanatomic mapping system has revolutionized the ability to perform activation mapping with rapid acquisition and automatic annotation of tens of thousands of electrograms from very narrow-spaced bipoles located on a 64 electrode mini-basket catheter. Human cases and detailed studies with this mapping system in large animal models of postinfarct VT have led to a reappraisal of much of the physiology of VT circuits. In particular, the central diastolic conduction isthmus, previously considered to represent the zone of slow conduction in this form of reentry, has been shown to have normal conduction velocity in most cases. The necessary conduction delay seems to occur because of wave front curvature around the functional barriers at the entrance to and exit from these isthmuses ( Fig. 30.9 )

Entrainment Mapping
In addition to confirming reentry as the arrhythmia mechanism, entrainment mapping allows for rapid and precise delineation of all the components of a reentrant VT circuit. This is true whether the conduction barriers that constrain the reentrant wave front are determined anatomically or functionally or both. The principles of entrainment were first described by Waldo et al. using the example of typical cavotricuspid isthmus–dependent atrial flutter following which they made similar observations in reentrant VT. These concepts were then extended by multiple other investigators to develop the criteria for entrainment mapping of VT circuit components.
Fundamentally, entrainment mapping seeks to answer two basic questions. Firstly, is a particular site located within the reentrant VT circuit and, secondly, is it within a narrow, constrained diastolic isthmus where the circuit may be vulnerable to interruption with ablation. These questions are answered at each site by analysis of the response to ventricular overdrive pacing ( Fig. 30.10 ).



After mappable VT is induced, the surface 12-lead QRS morphology is examined to obtain a first approximation of the likely region of reentrant wave front exit from the scar. Detailed analysis of the QRS morphology in various postinfarct VTs was first performed in the seminal intraoperative mapping study by Miller et al. Various combinations of surface ECG parameters that allow for VT localization are summarized in Table 30.1 . The roving mapping catheter is placed further back in the scar from the suggested exit zone where a systematic search is conducted for MDP. These may be recorded from the critical central isthmus or from adjacent bystander sites (where ablation would be ineffective). Entrainment mapping seeks to determine how such potentials relate to the VT circuit. The relative activation time of such potentials is much earlier than the presystolic potentials targeted in focal VT ablation. It should be noted that such very early activity may occur within the QRS complex of the preceding beat, particularly in very broad complex VTs where the QRS width is half or more of the VT cycle length. It is common for a larger far-field ventricular electrogram to be recorded along with an MDP.
Ecg Characteristic | Region of VT Exit |
---|---|
Bundle branch morphology
| Left ventricular septum or right ventricle Free wall of left ventricle |
Frontal plane axis
| Inferior or inferoseptal/lateral LV Anterior or anteroseptal/lateral LV Lateral LV or apex |
Precordial transition (R>S)
| Basal left ventricle Apical left ventricle Mitral annular Apex |
QRS upstroke
| Epicardial Epicardial |
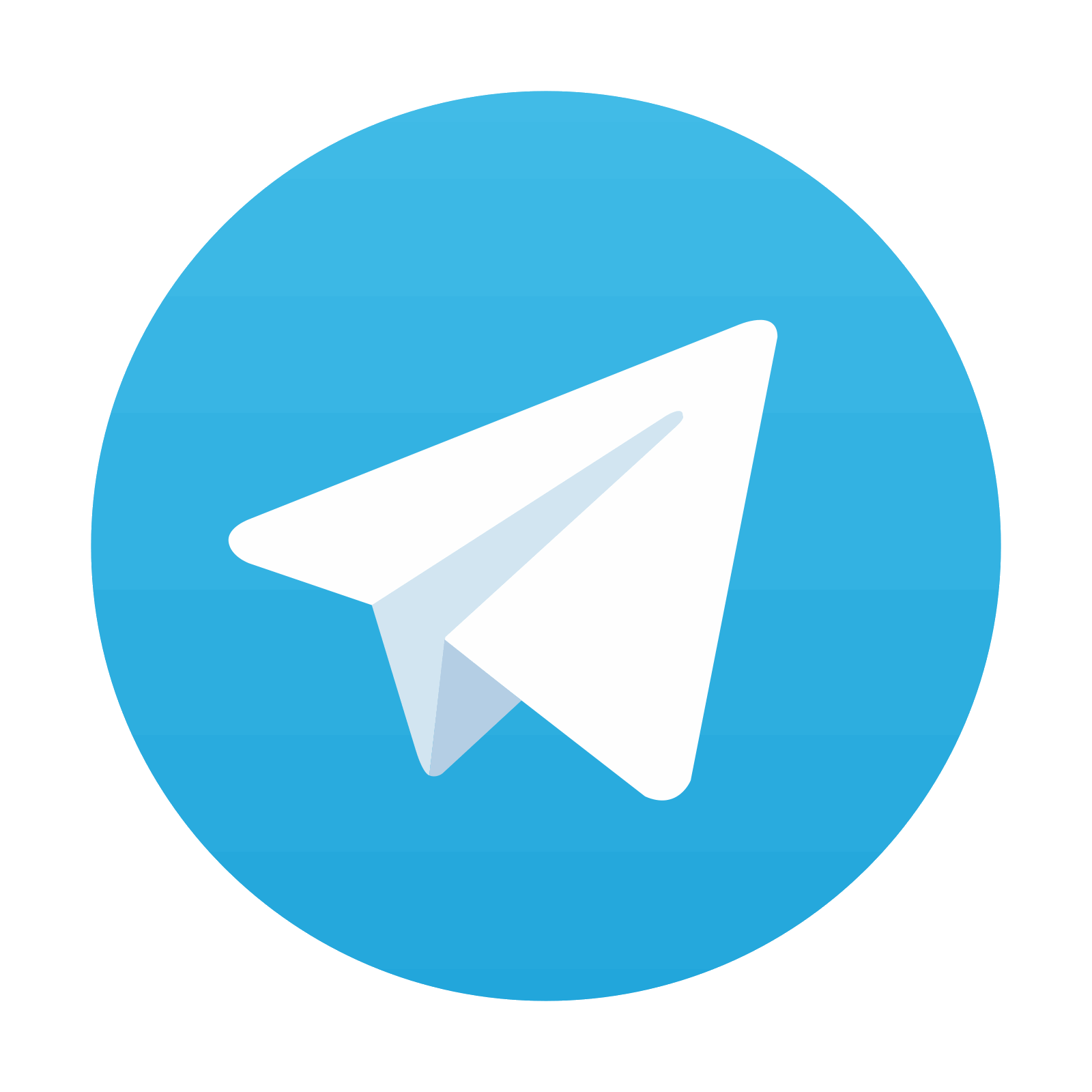
Stay updated, free articles. Join our Telegram channel
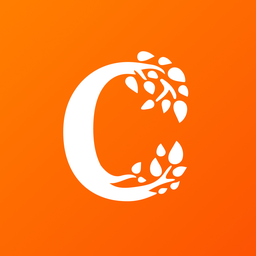
Full access? Get Clinical Tree
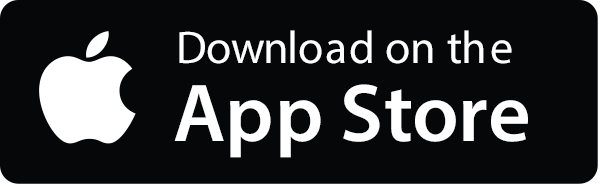
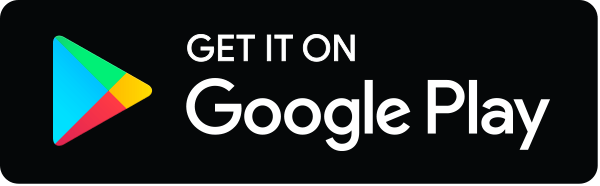
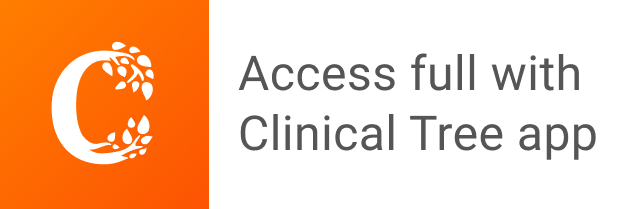