Definitions and Epidemiology
Acute respiratory distress syndrome (ARDS) is characterised by acute inflammation affecting the gas exchange surface of the lung, presenting clinically with acute hypoxaemia, in the presence of bilateral pulmonary infiltrates on chest radiography.
It has to be made clear from the outset that ARDS is not a disease. It is a state of dramatically diminished lung function of variable aetiology. A working definition of ARDS was established in 1994 by the American-European Consensus Conference. A more recent report recommends use of definitions of mild, moderate and severe ARDS, based on the degree of hypoxaemia (Table 44.1). Additionally, the exclusion of pulmonary oedema secondary to cardiac failure is now not mandated, positive end expiratory pressure (PEEP) is accounted for as an indicator of severity, and a known ARDS risk factor must be present within 7 days of onset.
Table 44.1 Berlin definition of ARDS
Mild ARDS | Moderate ARDS | Severe ARDS | |
---|---|---|---|
Timing |
| ||
PaO2:FiO2 | 201–300 mmHg | ≤200 mmHg | ≤100 mmHg |
PEEP | PEEP ≥ 5 cmH20 | PEEP ≥ 5 cmH20 | PEEP ≥ 10 cmH20 |
Chest X-ray | Bilateral opacities | Bilateral opacities | Bilateral opacities |
PaO2/FiO2 arterial partial pressure of oxygen/inspired oxygen fraction.
PEEP positive end expiratory pressure.
ARDS develops after exposure to a wide variety of insults, and given the nature of the diagnostic criteria, should be considered a syndrome rather than a disease. Initiating insults can be divided into two groups: direct (e.g. pneumonia) or indirect (e.g. sepsis) as outlined in Table 44.2. Risk of progression to ARDS varies according to the type, number and severity of predisposing conditions, as well as the genetics and other patient characteristics including gender, body mass index, smoking status, and alcohol usage. Diagnosing and treating conditions that mimic, or are associated with, ARDS is the first principle of successful management; prediction scores such as the Lung Injury Protection Score (LIPS) are intended to facilitate early recognition and treatment.
Table 44.2 Hallmarks of indirect versus direct ARDS
Indirect ARDS | Direct ARDS | |
---|---|---|
Causes |
|
|
Clinicopathological hallmarks |
|
|
Incidence and Outcomes
Data from the late 1990s determined that the age adjusted incidence of ARDS was 86.2 per 100,000 person-years, with an in-hospital mortality rate of 38.5%. Other data suggest that the incidence of ARDS may have declined since the 1990s. The decline in incidence has been attributed to improvements in healthcare delivery and process, including adherence to low tidal volume ventilation, and early recognition and management of sepsis. More recent data bring the reported declines into question, and suggest that ARDS occurs in 10.4% of all ICU admissions, with a hospital mortality of 34.9–46.1%, although these data are not without controversy.
Once treatment of the precipitating condition has commenced, prognostic factors relate to the patient’s response to therapy. Severe arterial hypoxaemia (PaO2/FiO2 < 100 mmHg) and an increase in the pulmonary dead-space fraction (>0.60) are associated with increased mortality. Mortality also correlates with the number of organ system failures, increasing to 83% when three or more are present. Complications appearing during the course of ARDS, including circulatory shock, acute renal failure and liver dysfunction, allied with age over 60 years, are associated with a higher mortality. Lung function in most survivors returns to normal over 6–12 months, although the majority have persistent, abnormal exercise endurance, and neuromuscular and neurocognitive morbidity significantly impairs longer term health related quality of life.
Pathobiology
Alveolar Epithelial Injury
The alveolar epithelium is composed of approximately equal numbers of flat type I cells (hAT1) and cuboidal type II cells (hAT2). hAT2 have several critical functions, including surfactant production, ion transport and functioning as progenitor cells for the regeneration of hAT1 cells after injury. Typical histological appearances of ARDS include extensive necrosis of hAT1 and the formation of protein-rich hyaline membranes on a denuded basement membrane. The extent of alveolar epithelial damage is a predictor of outcome.
Alveolar fluid clearance by hAT2 is primarily driven through sodium uptake on the apical membrane, followed by extrusion of sodium on the basolateral surface by Na+K+-ATPase. Loss of alveolar epithelial integrity and down regulation of sodium and chloride transporters results in the accumulation of protein-rich and highly cellular oedema fluid in the interstitium and alveoli. Loss of surfactant producing hAT2, allied with the effect of plasma proteins in the airspace, contributes to ARDS through atelectasis, increased oedema formation and impairment of local host defence. Clinically this presents with collapse of lung units and reduced pulmonary compliance. Epithelial cells also play key roles in regulating the inflammatory response through production of injury-driven pro-inflammatory cytokines and chemokines, the expression of leucocyte adhesion molecules, and cell–cell interactions with resident lung cells, particularly alveolar macrophages.
Endothelial Activation and Injury
The pulmonary endothelium forms a continuous barrier of endothelial cells, which regulate fluid permeability as well as modulating host inflammation, vascular tone, angiogenesis and interactions with blood-borne cells. Loss of barrier integrity, characterised by the formation of reversible intercellular gaps between endothelial cells, is accepted as the ultrastructural basis for the pulmonary oedema observed in ARDS. Gap formation is induced by the binding of mediators, including thrombin and tumour necrosis factor-alpha (TNF), which induce cytoskeletal rearrangement and endothelial barrier disruption.
Similar to the alveolar epithelium, the lung endothelium orchestrates and propagates the inflammatory response. Endothelial cells release cytokines and chemokines, up regulate the expression of adhesion molecules, and shift from an antithrombotic to a pro-thrombotic activated state, resulting in capillary thrombosis and extravascular fibrin deposition, potentiating pulmonary inflammation and contributing to the increased dead-space fraction observed in ARDS. Inflammation in the vascular space also counteracts hypoxic pulmonary vasoconstriction, partly by causing dysregulation of the production of vasoactive mediators including prostanoids, endothelins and nitric oxide.
Role of Circulating Cells
The recruitment of circulating inflammatory cells into the lung has long been recognised in ARDS. Neutrophils are central to the initiation and propagation of the inflammation observed, and neutrophilic alveolitis is a histological hallmark of ARDS (see Figure 44.1). The extent of neutrophilia present within the bronchoalveolar lavage fluid (BALF) of patients with ARDS has been reported to correlate with clinical outcome. However, the presence of neutrophils per se is not damaging, rather the priming/activation status of these cells is the major determinant of their subsequent injury-inducing behaviour. Recent data have shown that the healthy human pulmonary endothelium plays a role in host defence by trapping primed/activated neutrophils, facilitating their depriming, and later releasing them back into the systemic circulation in a quiescent state. Failure of this homeostatic depriming mechanism was observed in patients with ARDS.
Figure 44.1 Bronchoalveolar lavage fluid from a patient with ARDS. Photomicrograph of modified Wright’s stained cytospin. The white arrow denotes a hypersegmented neutrophil; the black arrow denotes a neutrophil which has been efferocytosed by a macrophage.
Monocytes appear to play a role in regulating neutrophil influx to the lung. However, whilst direct depletion of monocytes in mice consistently reduced LPS-induced blood and alveolar neutrophilia, as well as lung injury, peripheral mononuclear cell depletion in humans was unsuccessful in preventing the recruitment of neutrophils into the alveolar space.
Ventilator Associated Lung Injury
The application of mechanical ventilation exacerbates ARDS in a process called ventilator-associated lung injury (VALI). Mechanical forces applied during ventilation cause physical disruption of the alveolar-capillary membrane leading to pulmonary oedema, whilst cyclical stretch induces activation of cell- signalling pathways in epithelial, endothelial and inflammatory cells. In the context of the already injured lung, this results in pro-inflammatory and/or pro-fibrotic responses both locally and in the systemic circulation. Thus, in addition to local injury, VALI can drive systemic inflammation and extrapulmonary organ damage. This is reflected in the observation that the majority of patients with ARDS die from multisystem organ failure, rather than hypoxaemic respiratory failure.
Resolution of Inflammation and Repair of the Injured Lung
Most patients gradually recover normal physiology and lung function. In the alveolar epithelium, hAT2 cells proliferate in response to stimulation by epithelial growth factors. hAT2 cells are thought to act as progenitor cells for both daughter type II cells and type I cells. Local and bone marrow derived stem cells may also contribute to repair. Resolution of inflammation is macrophage and T-cell driven. Collectively, these mechanisms combine to reconstitute the epithelial barrier and restore lung function.
If epithelial injury is severe, or repair is impaired, a fibroproliferative phase of ARDS can ensue either following, or in parallel with, epithelial injury. During this phase, mesenchymal cells proliferate, neovascularisation occurs, and the alveolar space becomes filled with activated fibroblasts and myofibroblasts that synthesise excessive collagenous extracellular matrix. A small proportion of patients progress to a chronic phase of respiratory insufficiency characterised by widespread pulmonary fibrosis, with disordered lung architecture.
Clinical Investigations
The main clinical priority is identification and treatment of the initiating pathology and any complications that may have ensued. It may be necessary, particularly in the immune compromised host, to undertake invasive diagnostic procedures, such as bronchoscopy, to establish a diagnosis. Further, it has been shown that intercurrent pulmonary infection occurs in up to 70% of patients with ARDS, necessitating vigilance for the subsequent development of infection.
Ventilator Management
Protective Ventilation
The magnitude of the clinical burden of VALI was demonstrated by the ARDS Network study, in which patients with ARDS were randomised to receive either a high tidal volume (12 ml/kg predicted body weight (PBW)) with end inspiratory pressure limited to a plateau pressure Pplat ≤ 50 cmH2O, or a low tidal volume (6 ml/kg PBW) and Pplat ≤ 30 cmH2O. A PEEP ladder was used to determine the PEEP level administered, according to the fraction of inspired oxygen and the respiratory rate. The low tidal volume cohort demonstrated a 9% absolute reduction in mortality (40% to 31%). Accordingly, low tidal volume ventilation has become the standard of care in ARDS, and the goal of mechanical ventilation has shifted from normalisation of gas exchange parameters to minimising VALI with pragmatic acceptance of modest biochemical derangement.
High PEEP and Recruitment Manoeuvres
The application of PEEP has been used to mitigate the pulmonary oedema and atelectasis of ARDS. Several studies have failed to show clinical benefit of higher PEEP levels; nonetheless, a meta-analysis demonstrated improved survival in those with most severe physiological derangements (PaO2:FiO2 < 200 mmHg). Despite these data, consensus for this approach is lacking, particularly as response is heterogeneous and may be associated with lung hyperinflation and haemodynamic compromise. Determination of ‘optimal PEEP’ levels has thus far been elusive; calculation of driving pressure, estimation of transpulmonary pressures using an oesophageal probe and imaging techniques have shown promise but none have been assessed in prospective trials and cannot be recommended routinely.
Recruitment manoeuvres involving a transient increase in transpulmonary pressure are designed to promote reinflation of collapsed alveoli. A variety of techniques have been proposed, including graded incremental pressure increases, and a sustained high inflation pressure manoeuvre followed by a decremental reduction to an optimal PEEP level determined by dynamic analysis of flow-volume relationships, as well as image guided strategies. Whilst data support improvements in oxygenation, such manoeuvres are associated with complications, in particular haemodynamic compromise, barotrauma and exacerbation of existing air leaks; these appear to relate to the frequency of applied manoeuvres.
Ventilatory Modes
Despite inverse ratio ventilation (IRV) demonstrating no clear benefit when compared with conventional ventilator modes, the application of airway pressure release ventilation (APRV) in ARDS has been advocated by some centres. APRV utilises inverse ratio, pressure controlled, intermittent mandatory ventilation in patients with unrestricted spontaneous breathing to maintain alveolar recruitment and improve oxygenation, whilst limiting inflation pressures and sedation. Unlike IRV, APRV does not mandate paralysis and hence is an attractive mode. Nonetheless, APRV risks tidal hyper-inflation, increased transpulmonary pressures, and has shown no clear benefit over conventional modes. The use of high-frequency oscillatory ventilation (HFOV) to deliver small tidal volumes at high frequency with high mean airway pressures has been similarly tempered by two large multicentre trials demonstrating no benefit in one and increased mortality in the other. Accordingly, despite the theoretical mitigation of VALI, HFOV can no longer be recommended in ARDS, and conventional ventilator modes should suffice for the majority of patients. Where conventional modes fail to facilitate low tidal volume ventilation and acceptable physiology, extracorporeal gas exchange techniques should be considered in appropriate patients.
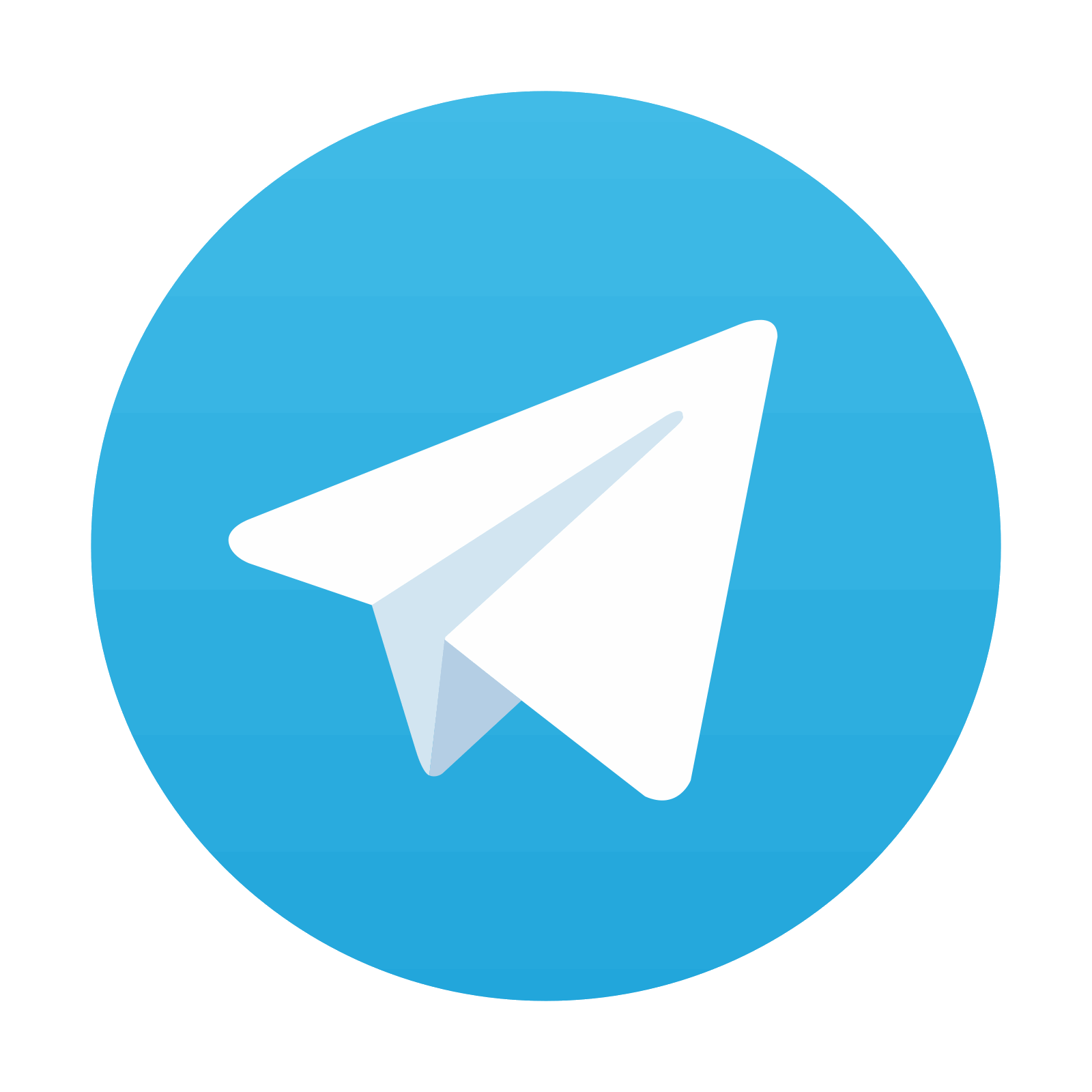
Stay updated, free articles. Join our Telegram channel
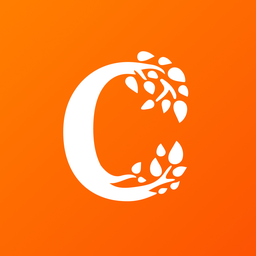
Full access? Get Clinical Tree
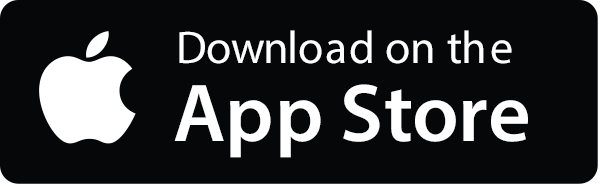
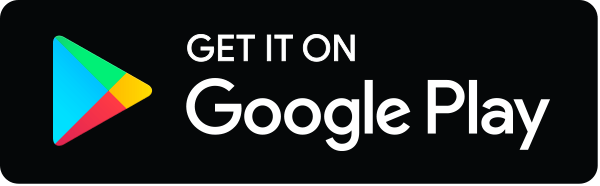
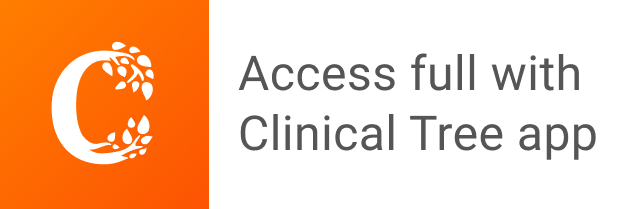