Fig. 18.1
Strategies to generate microvasculatures and vascularized constructs. a–d Self-assembly of cells for vascular grafts generation. a Sequential steps of cell fusion of vascular tissue spheroids in collagen I hydrogel [50]; b Bioprinted tubular structures with cellular cylinders [51] (a) design template, (b) bioprinter with two vertically moving print heads, (c) printed construct; c Fusion pattern of multicellular spheroids assembled into branched structure [51] (a) built of 300-μm spheroids with branches of 1.2 mm (solid arrow) and 0.9 mm (broken arrows), (b) fused branched construct after 6 days of deposition; d Built of a double-layered vascular wall [51] (a, e) multicellular cylinders assembled by SMC (green) and fibroblasts (red), (b, f) H&E staining, (c, g) α smooth muscle actin (brown), (d, h) Caspase-3 (brown). e–g Generation of microvasculatures by inkjet-based bioprinting [54]. e Schematic drawing of simultaneous deposition of HMEC and fibrin channel scaffold using modified thermal inkjet printer; f Printed fibrin scaffold; g Printed ring-shaped microvasculature, (a) cultured for 21 days (calcein AM, green), (b) improved integrity after 21-day culture with all dextran molecules (red) excluded from the printed structure. h–j Generation of bioprinted constructs with growth factor delivery [56]. h Cumulative release of VEGF. Fast (directly incorporated in Matrigel) and slow release (application of gelatin microspheres) of VEGF; i Bioprinted hydrogel mixture and tubulogenesis assay; j Vessel formation in EPC seeded scaffolds after one-week subcutaneous implantation in mice: (a) fast release showed less CD31 (brown) than (b) slow release of VEGF in the bioprinted hydrogels (Matrigel:alginate = 3:1). Blood vessels are indicated with arrows. k–n Coaxial nozzle-assisted 3D bioprinting of vasculature. k Schematic of fabrication of a 3D alginate structure with built-in microchannels [37]; l 3D construct fabricated based on hollow alginate filaments [37] (a) printed construct, (b) longitudinal section, (c) cross section, (d) SEM image of the cross section; m Formation of printed carbohydrate–glass filament architecture and vascular lumen with endothelial monolayer after removing sacrificial filament and perfusion [59]; n (a) Formation of dual channel by bioprinting sacrificial gelatin within fibrin, (b) GFP-HUVEC (green) within fibrin showed tube structure and capillary network after 12-day culture and RFP-HUVEC (red) in the channel-developed lumen structure [60]
Inkjet printing can also be used to bioprint vascular structures. A commercial inkjet printer has been modified to do just this. It was modified to simultaneously deposit human microvascular endothelial cells (HMEC) and fibrin to form microvasculature (Fig. 18.1e) [54]. The printed fibrin scaffold retained its proper printed shape (Fig. 18.1f), while the endothelial cells proliferated around it to form a tubular structure. The printed ring-shaped microvasculature had much better integrity after a 21-day culture, excluding Texas Red-conjugated dextran from the printed structure (Fig. 18.1g). This strategy shows that inkjet bioprinting has the ability to bioprint multiple cell types together, and that the use of mesenchymal stem cells (MSC) or smooth muscle cells can help support the formation and maturation of microvasculature. A problem with the method of inkjet bioprinting is that the cell deposition is performed on a substrate (biopaper) to support the weak cell layer(s).
Angiogenic growth factors play an important role in neovascularization. They signal cells to perform certain functions crucial to blood vessel generation. Angiogenic growth factors can activate endothelial (progenitor) cells and regulate their migration. They also promote cell assembly, vessel formation, and maturation [55]. The correct processes must be used to deliver the growth factors properly. For the best results, special delivery strategies, such as using nanoparticles and microspheres, are used instead of direct addition in vitro and injection in vivo. The controlled release of vascular endothelial growth factor (VEGF) from gelatin microparticles (GMP) within 3D bioprinted scaffolds and the effects on subsequent vascularization have been investigated [56]. VEGF was incorporated into Matrigel for fast release, or was put into GMP, which was then dispersed throughout Matrigel plugs for slow release. The application of GMP embedded in Matrigel plugs showed a significant, prolonged release of VEGF (Fig. 18.1h). Alginate was added to the Matrigel to enhance the mechanical properties and 3D printability. The problem with this addition was that large cell aggregates were induced and there was reduced tubulogenesis of endothelial progenitor cells (EPC) (Fig. 18.1i). A working combination of Matrigel and alginate mixture was found to be Matrigel/alginate = 3/1 with VEGF-incorporated. Heterogeneous 3D bioprinted scaffolds made of this mixture were subcutaneously implanted in mice. During this experiment, it was found that the slowly released VEGF promoted more vessel formation with more CD31 expression than the fast release VEGF (Fig. 18.1j). Nano-/microparticle delivery strategies influence the effectiveness of growth factors. The delivery strategies can enable dual or even multiple therapeutic reagents and angiogenic factors to be released in a synergistic way within bioprinted constructs [57].
All cardiovascular tissue requires a vascular network to maintain cell viability and to meet the oxygen and nutrient demands of the tissue. This is true of both micro- and macro-engineered tissue. The large, clinically relevant cardiovascular constructs must have flow throughout the entire construct. As useful as they are, angiogenic factors and endothelial cells are not able to provide immediate flow channels or generate perfusable constructs within a short time period [58]. This is where 3D bioprinting can help. 3D bioprinting provides the opportunity and ability to produce controlled vascular networks with clinically relevant size, perfusable channels, and multiple cells types. A coaxial nozzle-assisted 3D bioprinting system to fabricate hollow calcium alginate filaments has been implemented [36]. A sodium alginate solution, which can be cellular or acellular, is dispensed through the outer tube of the coaxial nozzle. This solution becomes cross-linked when it comes in contact with calcium chloride that is dispensed through the inner channel of the nozzle. The combination forms a filament with a hollow channel (Fig. 18.1k). The hollow alginate filaments were then used as the building blocks for further printing (Fig. 18.1l). Scanning electron microscopy image (SEM) confirmed the formation of a hollow structure and the uniform fusion section between adjacent hollow filaments (Fig. 18.1l). Other than directly printing the channel, it is also possible to create channel networks within engineered tissue constructs by printing sacrificial materials.
This sacrificial material process involves 3D printing networks out of an easily soluble material inside of a bulk material. This bulk material can be bioprinted or casted, and would typically be made of cell-laden hydrogels. The channel network is then dissolved or discarded by the method that best gets rid of the material chosen. For example, Miller et al. printed rigid 3D filament networks of carbohydrate glass. These were put inside of a fibrin hydrogel and then were sacrificed. The carbohydrate glass networks were used as cytocompatible sacrificial templates to generate cylindrical networks (Fig. 18.1m) [59]. Sugar-glass networks are compatible with many types of cell-laden matrices. The channel networks formed after removing the sugar-glass is able to support endothelial cells and withstand the pulsatile flow of human blood. They even have intervessel junctions to support branched fluid flow (Fig. 18.1m). A bioprinted template of agarose fibers has been utilized to fabricate perfusable microchannel networks within gelatin-based hydrogel constructs [39, 40]. The fabricated networks improved mass transport, cellular viability, and differentiation of cells within the cell-laden constructs. A process has also been developed in which two fluidic vascular channels are deposited with a fibrin-cell mixture in the middle (Fig. 18.1n) [60]. Human umbilical cord vascular endothelial cells (HUVEC) transfected with green fluorescent protein (GFP, green) and mCherry (red) were separately cultured and used for fibrin gel and fluidic channels, respectively (Fig. 18.1n). The HUVECs began to form tubular structures after a 1-week culture, and the capillary network became denser, creating more branches with a lumen. Both Pluronic F127 and methacrylated gelatin cell-laden hydrogels have been printed as sacrificial materials to form perfusable networks [61]. Four print heads were used in this process: first, a PDMS border was printed, then the sacrificial Pluronic F127 with two different Gel-MA inks containing fluorescent labeled fibroblasts. Pluronic was removed, and the microchannels were endothelialized with RFP HUVEC.
3D Bioprinting of Myocardium
Within the realm of CVD, ischemic disease (e.g., myocardial infarction (MI) or a “heart attack”) is the leading cause of death. Ischemia is the lack of oxygen delivery to the heart. Heart attacks represent 42% (7.3 million) of all CVD deaths [62]. An acute heart attack is normally caused by the blockage of one of the coronary arteries, which results in a lack of blood flow and ischemia. When deprived of oxygen, cardiomyocytes (CM) die and a vigorous inflammatory response is incited. This causes fibroblasts and endothelial cells to migrate to the area and form noncontracting fibrotic scars. Scars contain little myocardial tissue and reduce the contractile function of the heart, ultimately leading to heart failure. During injury, a great number of CM die. Due to the relative inability of CM to divide, these lost cells are not replaced [63]. In severe cases of heart failure, heart transplantation is often the next consideration in treatment. This strategy is complicated and not always ideal due to a shortage of donor organs and the risk of organ rejection by the body. Cellular cardiomyoplasty, or cell-based cardiac repair, has made remarkable progress in myocardial tissue regeneration [64]. This approach involves injecting cells into the myocardium, a less invasive procedure than other treatments. This technique has been limited by the low viability and difficulty the injected cells have in integrating with the host cells.
Tissue engineering and bioprinting might be able to help with cell survival and growth. Myocardial tissue engineering (MTE) requires the use of a high density of CM and other supporting cells, along with proper vascularization and efficient oxygen exchange, in order to generate synchronous contractions [65]. 3D bioprinting can be used to pattern and assemble cells with high density, defined organization, and defined spatial distribution. It also allows the creation of multilayered constructs with multiple cells types within each layer. Laser-induced inkjet bioprinting has been used to pattern HUVEC and human mesenchymal stem cell (hMSC) on a polyester urethane urea cardiac patch (Fig. 18.2a) [66]. It is possible to generate specific vascular patterns with HUVEC (green) and hMSC (red) arranged in a capillary-like pattern (Fig. 18.2b). Some patches with patterned cells and some with randomly seeded cells were transplanted in vivo to the infarcted zone of rat hearts after left anterior descending (LAD) ligation (Fig. 18.2c). EBB has been used to bioprint alginate and RGD-modified alginate scaffold with human fetal CM progenitor cells (hCMPC) (Fig. 18.2d) [67]. It was demonstrated that within the 3D culture, the printed hCMPCs had high cell viability (Fig. 18.2e). This group further implanted the bioprinted patch composed of an hCMPC HA/Gel matrix in a mouse model of myocardial infarction [68]. In this test, the hCMPCs retained their cardiogenic phenotype in the bioprinted constructs for up to one month (Fig. 18.2f, g). The patch was able to preserve heart function by reducing LV remodeling and improving myocardial viability.


Fig. 18.2
3D bioprinting of myocardium. a–c Inkjet-based bioprinting of cardiac patch [66]. a Schematic bioprinting setup, b Patterned cells, c Patch implantation in vivo in a rat model. d–g Extrusion-based bioprinting of scaffolds with hCMPC d, e [67], f, g, [68]; d Bioprinted scaffolds, e High cell viability of hCMPC, f in vivo engraftment of the printed patch on the infarcted area of the ventricular wall for 4 weeks, g Presence of human Lamin A/C
As in standard MTE approaches, it is essential for 3D bioprinting of thick muscle-like tissues to generate synergistic contractile force. This is essential for the adequate repair or replacement of damaged heart tissue. One problem is the difficulty to functionally integrate the graft and the host tissue. This problem is due to both electromechanical and vascular connections. The cells being used in the 3D bioprinting of vascular tissue are stem cells, such as embryonic stem cells (ESC) and induced pluripotent stem cells (iPSC). Autologous cells, such as skeletal myoblasts and mesenchymal stromal cell (MSC), are also being utilized yet are not very successful at integrating with host cardiovascular tissue. The ideal cell source does not seem to exist [69]. This means that different strategies will need to be developed to use the cell sources available in a more effective manner. 3D bioprinting has the ability to control the micro- and macro-architecture as well as the pore size/porosity, but the challenge of vascularizing thick tissue constructs has not been able to be overcome as of yet.
3D Printing of Heart Valves
The human heart has four valves that ensure unidirectional flow of blood. There are two atrioventricular/inflow valves, known as the mitral and tricuspid valves, and two semilunar/outflow valves, known as the aortic and pulmonary valves. Each valve is composed of leaflets that are attached to a fibrous annulus wall or root wall. Both the leaflets and root wall are biomechanically and structurally anisotropic [70, 71]. Leaflets and root walls are made up of mostly valve interstitial cells (VIC) and smooth muscle cells (SMC), respectively. They have valvular endothelial cells (VEC) covering the surfaces. The pathophysiology of valve disease is broad. One of the most common heart valve abnormalities is calcific aortic valve disease (CAVD) [72]. CAVD is commonly treated with surgical or interventional repair or replacement. The options for these replacements are mechanical or bioprosthetic valves [73]. Tissue engineering has great potential to address current limitations of nonliving prosthetics by providing living constructs that can grow, remodel, and integrate into the patients.
3D bioprinting, mainly EBB, has been implemented in the fabrication of tissue-engineered heart valves. The advantages of using 3D bioprinting techniques over traditional tissue engineering approaches are the ability to generate anatomically accurate trileaflet valves, mechanically heterogeneous valve conduits, and living engineered valves with correct spatial and temporal valve cells (VIC and SMC) distribution.
There are several different valve models and designs that have been used for 3D bioprinting. Duan et al. implemented a flat model with bioprinted trileaflet valve conduits. They used a combination of methacrylated hyaluronic acid and methacrylated gelatin to accommodate human aortic VIC (Fig. 18.3a, b) [74]. Control of the hydrogel viscosity and construct stiffness was created by optimizing the polymer ratio and concentration. The encapsulated cells were shown to have high viability and matrix remodeling ability with sulfated GAG deposition (Fig. 18.3c). This testing model did not contain any sinuses, which are the widenings between root wall and leaflets. Both of these structures are needed to relieve abnormal stress and prevent blood back flow. Hockaday et al. used an improved design with an axially symmetric shape (Fig. 18.3d, e) and a combination of 700 and 8000 MW poly(ethylene glycol) diacrylate (PEGDA) to print valve conduits with biomechanical heterogeneity. In this design, the leaflets were more flexible while the root remained relatively rigid [75]. Axially symmetric heart valve scaffolds can be printed with various dimensions, showing that they can be made to fit adults and children, as well as varying heart sizes (Fig. 18.3f). The same group also was able to create an aortic valve geometric model using a μCT of a porcine aortic valve that was freshly obtained (Fig. 18.3g) [76]. When used with the scan, the model was able to maintain the normal anatomical characteristics of native valves, such as the ostium and sinus (Fig. 18.3h–j). For heterogeneous bioprinting, the valve root, made of SMC laden hydrogel, was deposited first and subsequently, the leaflet region of the layer, made of VIC-laden hydrogel, was extruded along its print paths (Fig. 18.3k) [77]. The same steps were repeated with aortic valve conduits with SMC and VIC encapsulated in the root and leaflet tissues, respectively. These exhibited geometry comparable to the original image of the derived valve (Fig. 18.3l, m) [77].


Fig. 18.3
3D bioprinting of heart valve. a–c Flat valve [74]; d–f Axisymmetric valve [75]; g–m Anatomical valve [77]. a, d, e, k Valve model; b, f, m Bioprinted valve; c Safranin-O staining showed GAG deposition; h μCT scan slices and their reconstruction; i, j The valve scans were viewed, thresholded, and segmented into separate STLs for the leaflet and the root; l Fluorescent image of first printed two layers of aortic valve conduit
Tissue-engineered valve size and geometry play important roles in maintaining functionality and stability under hemodynamic loading conditions [78, 79]. Computer simulations show that incorrect valve design may result in radial compression and eventually reduces leaflet size [80]. It is not known yet how valve geometry influences hemodynamic properties or how cells in the bioprinted valve conduits respond to such changes and remodel the matrix.
3D Bioprinting for Pediatric Applications
Deaths in children due to congenital heart disease, structural or electrical cardiac abnormalities, rank sixth in causes of death in 1- to 19-year-olds [81]. Cardiovascular repair or regeneration for children poses numerous challenges due to the small patient size, the risk of thromboembolism, the highly dynamic environment, and immature cell populations present in pediatric patients. In addition, the cardiovascular tissues in young patients are growing and remodeling much faster than in adults. Therefore, many prosthetic devices are suboptimal options because they cannot grow, remodel, or integrate with the host tissue [82]. 3D bioprinting allows for more precise fabrication of living constructs than traditional techniques. Hydrogel valves have been printed to span a range of clinically relevant sizes, as small as 12 mm inner diameter, which is approximately the size of a 6-month infant aortic valve, up to 22 mm inner diameter, which is an adult size [75]. The shape fidelity of printed valves was characterized using surface deviation analysis through micro-CT imaging in comparison with the design (Fig. 18.4a). In general, the accuracy of the design decreased with the decrease in the valve size, but the overall shape fidelity was decent even for pediatric valve printing. Recently, a whole heart structure was created using a thermoreversible support bath to enable bioprinting of freeform reversible embedding of suspended hydrogels [83]. This process allowed the printing of embryonic hearts with mechanical robustness and complex 3D internal and external anatomical architectures at a resolution of ~200 μm (Fig. 18.4b–f). Several groups have been using decellularization techniques to decellularized embryo or fetal tissues [84, 85]. It has been demonstrated that fetal cardiac ECM significantly promoted the adhesion and expansion of neonatal cardiomyocytes compared with neonatal ECM and adult ECM [86]. Therefore, decellularized fetal or neonatal cardiovascular tissues have great potential to be further processed into bioinks for bioprinting.


Fig. 18.4
3D bioprinting for pediatric applications. a Heat maps and average % frequency histograms representing surface deviations between printed porcine scaffolds with different sizes versus model geometries [75], b–f Printed whole heart based on 3D imaging data from chick embryo [83]; b Image of explanted embryonic chick heart; c 3D image of the embryonic chick heart stained for fibronectin (green), nuclei (blue), and F-actin (red); d Cross section of the 3D CAD model of the embryonic heart; e Cross section of the 3D printed heart (fluorescent alginate green); f 3D printed heart with internal structure visible through the translucent heart wall
Challenges and Future Perspectives
3D bioprinting is a promising technique for the generation of cardiovascular tissue due to its ability to print heterogeneous and clinically relevant-sized tissue constructs. Despite the great progress and promise, there are still many challenges that hinder its further applications.
Development of High-Performance Bioinks and High-Resolution Bioprinter
Although there are a large variety of bioinks to choose from, the challenge remains to develop the ideal bioink which is capable of printing biologically functional and mechanically robust tissue constructs. There are also many different tissue types, with varying properties, which makes finding the best bioink very difficult. The ideal bioink should be bioprintable, support cardiovascular cell functions, have comparable mechanical properties to native tissue/organs, and be affordable and commercially available with regulatory guidelines for clinical use [43]. One of the major hurdles in currently available hydrogel-based bioinks is to balance the cell functions and mechanical properties of cross-linked hydrogels. A high concentration of hydrogel results in stable mechanical properties, but causes low mobility and less spreading of the encapsulated cells. To get past this problem, bioinks that are crosslinkable in situ with spatially and temporally controllable cross-link rates and degrees are being developed [87, 88]. In addition, advanced biofabrication techniques are desirable to fabricate scaffold-free cell aggregation-based bioinks in high throughput to decrease the fusion time and enhance the mechanical properties and maturation.
Currently, a number of bioprinters, mainly extrusion-based, have been developed and commercialized. The commercialization of some bioprinters is limited due to the inadequate bioinks available. The ideal bioprinter should have at least a submicron resolution. It needs to have the ability to bioprint a matrix with an orientation which can induce the alignment of cardiovascular cells, such as cardiomyocytes and VIC. The bioprinter should also have multiple printing cartridges available to be used at the same time. This would allow the bioprinter to utilize multiple bioinks toward the bioprinting of one heterogeneous construct. This is especially significant for the bioprinting of the whole heart. More powerful and easy-to-use software would be needed to control the small resolution and multiple printing cartridges needed for the ideal bioprinter.
Bioprinting of Functional Cardiovascular Constructs
With 3D bioprinting being in its infancy, so are most of its applications, especially in the cardiovascular realm. A major problem with bioprinted constructs is the lack of mechanical strength and integrity. This is mostly due to weak mechanical properties of the hydrogel-based bioinks that are being used [89]. For heart valves, the tensile and compressive properties of different bioprinted constructs were found to be much smaller than the peak moduli of native valve tissue [74]. However, healthy valve cells are subjected to physiological strain range rather than failure strain. Within a normal physiological strain range (~15% strain), valve cells can behave and function normally. The stiffness and moduli of bioinks are tunable and quite comparable to those of pulmonary valve leaflets in the physiological strain range. Although hydrogel bioinks may not meet the full mechanical strength range of native valve tissue initially, the tissue-engineered constructs may be strengthened through further hemodynamic conditioning via collagen deposition and scaffold remodeling. Full characterization of the mechanical properties and functions of the constructs is crucial to produce the constructs that will withstand the complex hemodynamic pressures and flows of the cardiac environment [90, 91]. These properties and functions include electrophysiological functions, hydrodynamic response, compliance, and remodeling. In addition to having proper anatomical architecture and mechanical support, the bioprinted cardiovascular tissues should avoid thrombogenesis and be resistant to calcification after implantation. This is of particular importance for in vivo and pre-clinical applications. Avoiding these unfavorable developments often relies heavily on choosing and/or developing appropriate bioinks [92]. Another major point of importance for bioprinting cardiac tissue is to regulate the microenvironment, including matrix components, stiffness, and physiochemical stimuli. Regulating the microenvironment allows the control of the differentiation of stem cells and other cell sources toward the desired cardiovascular phenotypes [93].
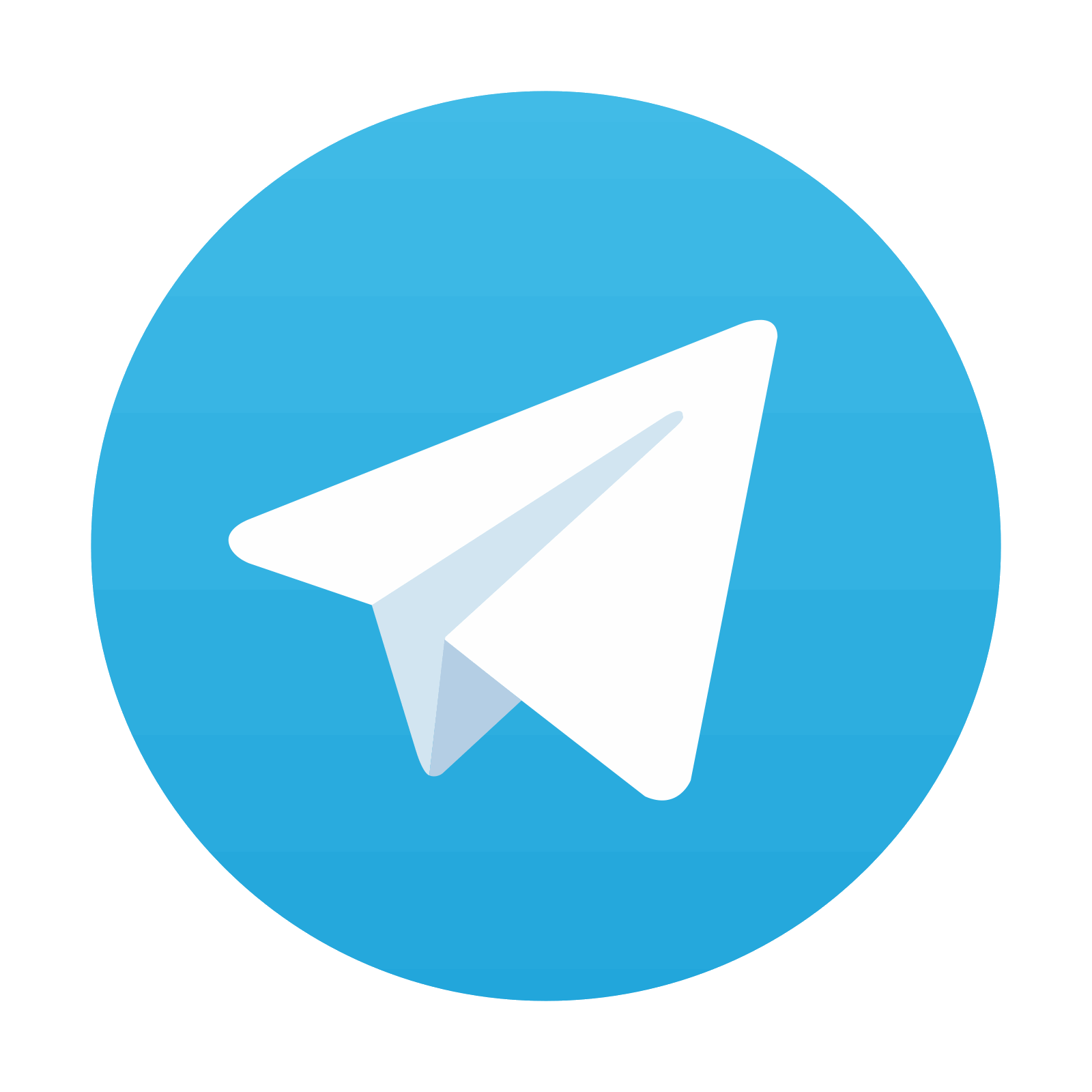
Stay updated, free articles. Join our Telegram channel
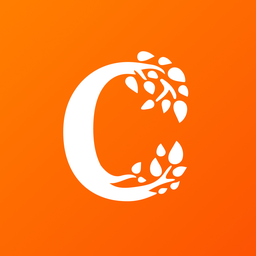
Full access? Get Clinical Tree
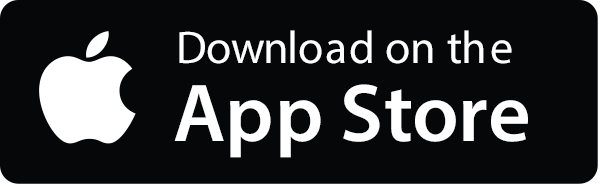
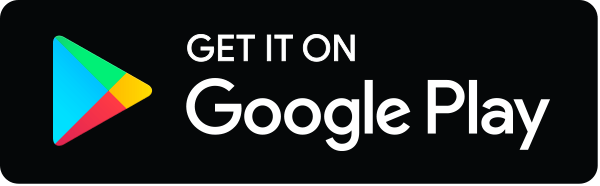