Keywords
MicrocirculationTypes of capillariesVascular endotheliumCapillary fluid dynamicsRevised Starling principleInterstitial spaceOncotic pressureNegative interstitial pressureOrthogonal polarization spectral imagingSidestream dark-field imagingCardiopulmonary bypassCritical illnessFluid therapyFunctional capillary densityCoherence between macro- and microcirculationHaving explored the heart and several aspects of the central circulation, we will now consider its opposite, the microvascular periphery. The microcirculation (MC) is a complex network of conduits adapted for exchange of respiratory gases and nutrients. Its large total surface area allows for greatest possible contact between the blood and the tissues. Each segment of the MC is unique in possessing functional and morphologic features adapted to the organ it supplies. Unlike the macrocirculation that is at the core of the pressure-propulsion model, the microcirculation has traditionally been ascribed only a secondary role. With the advent of new methods for in vivo monitoring of the microcirculatory function, this view has radically changed. Direct visualization and ways to quantify microvascular perfusion have uncovered its fundamental hemodynamic role in health and critical illness. Microvascular research has become the “new frontier” in cardiovascular medicine.
21.1 Morphology and Function
We have traced the origin of the cardiovascular system to the common, mesoderm-derived progenitors with the potential to become the heart, the vessels and their content, the blood (Chap. 1). This threefold differentiation is of far-reaching importance for the fate and function of its individual components. With the potential to transform into a vein or an artery, the capillary emerges as the basic building unit of the CVS. It will be recalled that the heart, too, originates from a capillary-size tube (cf. Chap. 12). It has long been recognized that not only the heart, but also the microvascular beds are subject to rhythmical oscillation known as spontaneous vasomotion , a phenomenon well described in vivo and in vitro [1, 2].

Microvascula r bed with schematic of its components (inset, below). A typical microvascular loop consists of terminal arteriole branching into metarterioles and a network of capillaries. Arterioles and venules are surrounded by a circular layer of innervated smooth muscle. Metarterioles are shorter, have a discontinuous muscle layer and lack innervation. Precapillary sphincters consist of a single cuff of smooth muscle that responds to local vasoactive metabolites. Pericytes play an important role in microvascular differentiation, angiogenesis, and paracrine signaling

Three types of capillaries. In continuous capillaries, the basement membrane and endothelial cells provide an uninterrupted lining allowing only water and ions to pass through the tight junctions (left). The endothelial cells in fenestrated capillaries have larger pores that allow free exchange of water, solutes, and large molecules (middle). In discontinuous or sinusoidal capillaries, the endothelial cells form loose junctions and contain large pores (30–40 μ in diameter), allowing for free passage of macromolecules and some cellular elements, e.g., red blood cells and lymphocytes
Continuous capillaries are found in the lung, heart, skeletal and smooth muscle, as well as in the subcutaneous and adipose tissue. They have a continuous basement membrane with narrow endothelial intercellular junctions. The brain capillaries present a special case in that the endothelial cells are fused to each other by continuous tight junctions which prevent direct passage of water and solutes via paracellular route. A thick basement membrane and a dense packing of astrocyte foot-processes offer an effective wall, i.e., the blood–brain barrier, that protects the brain cells from fluctuating osmotic and ionic currents of the blood. Of note, the brain capillaries offer 100–200 times higher electrical resistance than the capillaries in other parts of the body [8].
Fenestrated capillaries have endothelial cells interrupted with fenestrations measuring 0.005–0.0065 μm, some of which are covered by a thin membrane (0.004 μm). They are found in organs involved in active exchange with the surroundings, such as the gut mucosa, endocrine glands, ciliary body, glomeruli, renal medulla (vasa recta), and the choroid plexus in the ventricles of the brain.
Sinusoidal capillaries have a perforated basal membrane and endothelial cells with large temporary or permanent gaps (0.1–1 μm) that allow for exchange of macromolecules and cells. They line the sinusoids of the bone marrow, liver, and spleen.
From a functional perspective, the three types of capillaries point to the threefold structure of the organism mentioned in Chap. 12. According to this paradigm, the brain, virtually bereft of interstitial tissue and isolated from direct influences of the blood, is assured a stable electrochemical environment that supports its essential functions, namely, sensory and thought processing and the maintaining of consciousness. In contrast, the discontinuous capillaries of the metabolic pole allow for extensive exchange between the blood and the organs.
21.1.1 The Vascular Endothelium
The endothelial cells line the entire vascular tree from the heart to the smallest capillary and, with a total weight of over 1 kg and a surface area of up to 7 m2, form a substantial organ [9]. The vascular endothelium provides many important functions. For example, it plays an important role in maintenance of blood fluidity by inhibiting several components of the coagulation cascade. It moreover controls vessel wall permeability and is a key component in the matching of blood flow with metabolic demands of the tissues. The luminal surface of endothelial cells secretes hairy projections, the endothelial glycocalyx (EG), a gel-like structure whose main components are glycoproteins and proteoglycans consisting of a protein core and negatively charged glycosaminoglycans (GAGs) [10]. The EG forms a luminal mesh that binds plasma proteins and soluble GAGs and provides a dynamic barrier between the flowing blood and the endothelium. The negatively charged EG controls protein binding and vascular permeability by repelling negatively charged molecules, such as albumin, as well as negatively charged cellular components: RBCs, white blood cells, and platelets. The EG is a dynamic, fragile structure that maintains the integrity of the vascular barrier. It is highly sensitive to oxidative stress (reactive oxygen species) and its injury leads to several pathological conditions, including capillary leak, reduced vascular responsiveness, inflammation, platelet aggregation, and hypercoagulable states [11]. The endothelial cells moreover produce nitric oxide (NO)—a crucial molecule controlling the tone of the vascular smooth muscle—and prostaglandin (PGI2) which modulates platelet function and inflammatory response [12].
21.1.2 The Revised Starling Principle

Classic Starling principle of the capillary fluid dynamics. The sum of capillary hydrostatic and tissue oncotic pressures forces fluid out into the interstitial space at the arterial end of the capillary. At the venous end the sum of tissue hydrostatic and oncotic pressures causes net absorption of fluid into the capillaries (a). The model predicts a linear relationship between the sum-total of hydrostatic and colloid osmotic forces (b). + denotes positive intraluminal pressure; − negative interstitial pressure

Microvascular fluid exchange according to Starling (a) and endothelial filtration fluid models (b). Schematic assumes a continuous type of capillary. In the conventional Starling’s model, the endothelial layer is considered a semipermeable membrane that separates oncotically active protein particles between the capillary lumen and the interstitium (a) (long arrows). In the revised model, the endothelial glycocalyx assumes this role. The oncotic gradient is determined by a low protein concentration in the sub-glycocalyx layer (short arrows), resulting in reduced amount of filtered plasma (b). Endothelial glycocalyx also acts as a mechanotransducer to luminal sheer stress/pressure, causing release of nitric oxide (NO) by the endothelial cells and activation of aquaporins (cf. Sect. 15.2) which actively regulate water and small solute flux across the capillary wall
21.2 Metabolic Control of Tissue Perfusion
The physiological processes that regulate optimal matching between the metabolically active tissues and the supply of oxygen by the blood have traditionally been based on the oxygen transport model by August Krogh [16]. Krogh proposed that the amount of available oxygen is proportional to oxygen tension in the capillary and inversely proportional to its diffusion rate in the surrounding tissues. Based on the premise that the capillary is at the center of a tissue cylinder consuming oxygen at a constant rate, Krogh’s model predicts a linear drop in oxygen tension along the capillary. It should be noted that Krogh encountered considerable technical difficulties when estimating capillary densities in muscles of different animal species. The number of capillaries depends on the muscle type, e.g., limb or ocular muscle; on the thermal condition of the animal, i.e., cold blooded amphibians have fewer capillaries and much larger red blood cells than warm blooded mammals; and on the size and activity of the animal, such as in a rodent versus a horse. Unlike the parenchymal organs that readily take up contrast media (gelatin stained with Prussian blue) in vivo, the muscles are poorly perfused, hence the capillary counts were done on deceased specimens. As noted by Krogh, “The best results have been secured by injecting directly into the artery, tying as far as possible all the arterial anastomoses and employing high pressure” [16]. In addition to the abovementioned factors, Krogh’s model assumed that oxygen delivery to the tissues ultimately depends on capillary “recruitment” by the perfusion pressure at the level of the macrocirculation.

New methods of in vivo microvascular flow imaging unravel fundamental connections between local metabolic demands and perfusion. Representative image of in vivo mouse brain obtained by single red blood cell flowoxigraphy (single RBC FOG) shows heterogeneity in red blood cell O2 saturation within a small segment of tissue (a) (yellow rectangle, scale bar 200 μm). FOG can detect single RBC oxygen and hemoglobin concentration and velocity at millisecond time intervals and micrometer-scale spatial resolution as it traverses the capillary (b). The method has unique capability of quantifying neuronal metabolic activity and matching it with oxygen delivery with pinpoint accuracy. Color scale on the right of panel (a) shows relative oxygen concentration from 0 (blue) to 1 (red), and between 0.25 and 0.35 (b). (Reproduced from ref. [23], courtesey of PNAS)
21.2.1 Erythrocytes as Sensors and Controllers of Microvascular Perfusion
Collectively, the erythroid progenitors in the bone marrow and the RBCs in the peripheral blood are known as the erythron, to convey the idea that they function as a single organ [24] (cf. Figs. 1.2 and 12.2). The normal erythrocyte is a biconcave disc filled with a concentrated solution of hemoglobin with a diameter of 7–8 μm. The RBCs are marked for exceptional deformability that allows for passage through 5–10 μm-sized capillaries including 1-μm wide endothelial slits in the red pulp of the spleen [24]. Reversible deformation of the RBCs depends on its geometry, hemoglobin concentration, and viscosity of the protoplasm, and is essential for its function and survival. The unique elastic properties of the membrane results from the interaction between the outer lipid bilayer and of the inner, two-dimensional cytoskeletal network of flexible spectrin molecules, cross-linked with actin filaments and other structural proteins. Observed under a phase contrast microscope, the RBCs display characteristic thermally excited undulations of the membrane known as red cell flicker [25]. The membrane shape fluctuations and integrity are active, energy-dependent processes that depend on adequate supply of ATP and optimal (body) temperature [25, 26].
Since mature erythrocytes lack the nucleus1 and other cellular organelles, such as the mitochondria and endoplasmic reticulum, they depend for sources of energy entirely on (anaerobic) glycolysis, a remarkable adaptation, given the fact that they provide oxygen to all other tissues of the body.
The ability of vascular beds to meet and sustain metabolic demands of the tissues is known as vascular autoregulation . Multiple mechanisms have been identified which contribute to changes in vascular tone, such as intraluminal pressure (myogenic response), local metabolite concentrations, and the effect of shear stress on endothelial lining, to name a few. Increasing evidence suggests that local biochemical and mechanical factors work in conjunction with a key blood component, the erythrocytes. While a close relationship between the tissues’ need for oxygen and the cardio-respiratory response has been recognized for well over a century, the mediator or the “sensor” in this demand–supply loop has been one of the great physiological unknowns, until the active role played by the RBCs was demonstrated by Ellsworth and coworkers in the 1990s (Sect. 17.1.1) [28]. It turns out that rather than being passive “containers” for oxygen delivery, the RBCs actively regulate tissue oxygen supply and demand.

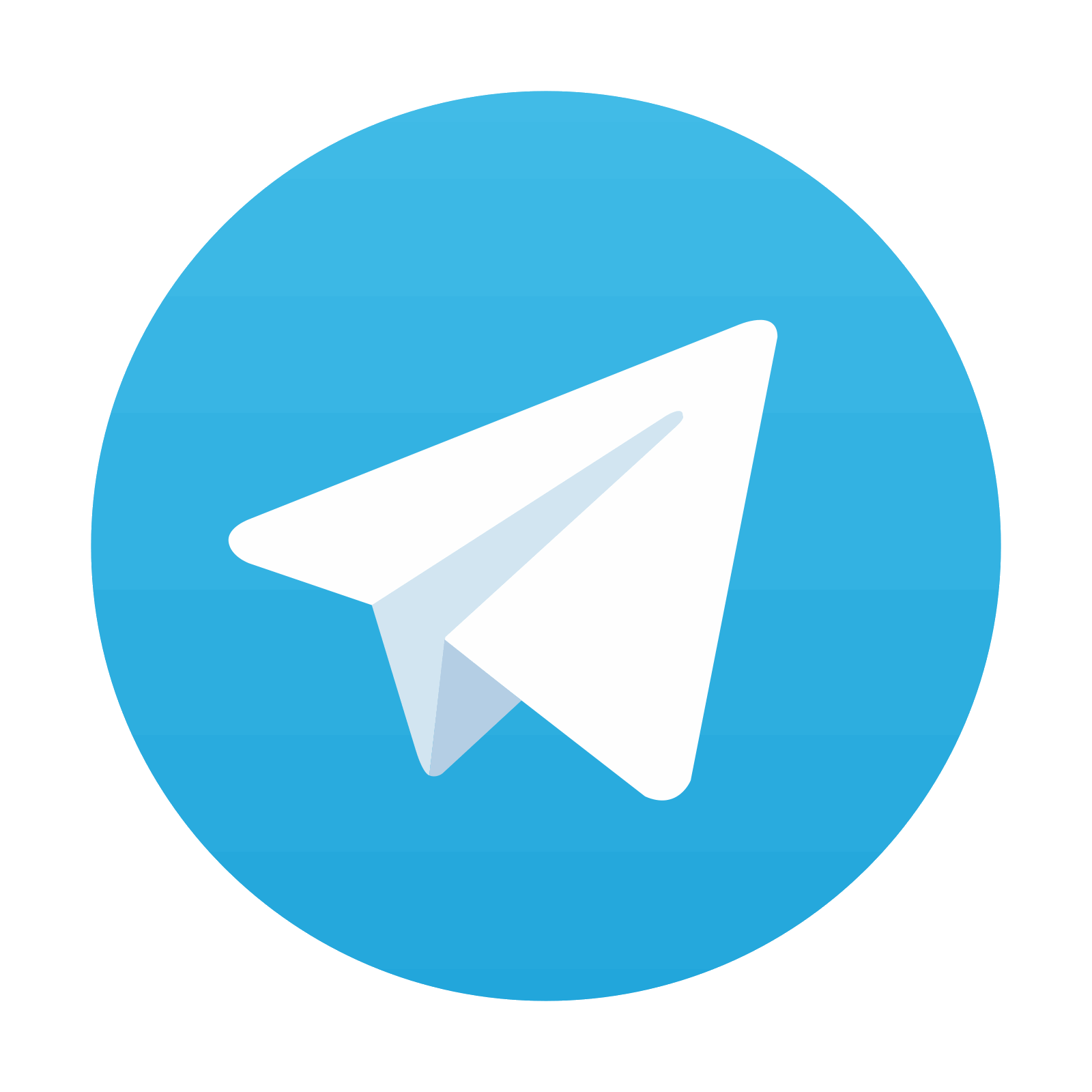
Stay updated, free articles. Join our Telegram channel
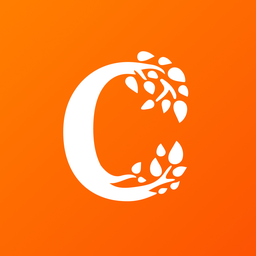
Full access? Get Clinical Tree
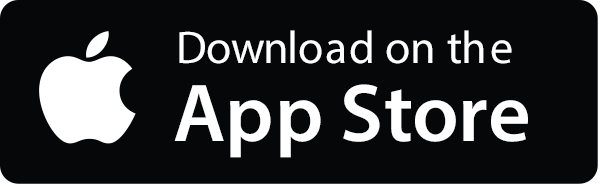
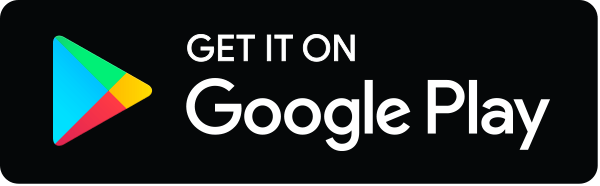