Simplified schematic view of the ventral mammalian brain stem. Rhythm generation resides mainly in the pre-Bötzinger (pre-BötC) complex, while pattern formation resides more caudally in the ventral respiratory column. CO2 chemosensitivity extends within the orange-shaded area beyond the traditionally accepted chemosensitive areas, while the nucleus tractus solitarius (NTS) receives peripheral afferents from IX and X cranial nerve afferents (XII = efferent hypoglossal fibers).
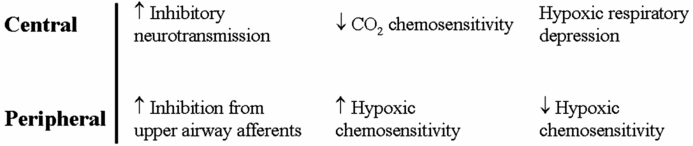
Central and peripheral mechanisms that contribute to instability of respiratory control in fetal and early postnatal life.
The primary rhythm-generating and pattern-forming network is rostrocaudally organized along the entire length of the ventrolateral medulla, forming bilateral columns of interconnected respiratory nuclei called the ventral respiratory columns (VRC). The pre-Bötzinger complex (pre-BötC) of the ventrolateral medulla is the main source of respiratory rhythm generation (3). The pre-BötC is a core group of synaptically coupled neurons that possess autorhythmic or pacemaker-like activity. Intrinsic cellular properties of the pre-BötC enable the neurons to generate a rudimentary pattern of inspiratory activity when experimentally isolated in vitro (4). In the intact brain stem, however, generation of inspiratory rhythm is much more complex. The role of the pacemaker-like cells is diminished by the rhythmic inhibitory, tonic excitatory, and other modulatory inputs that converge on the pre-Bötzinger complex. Unfortunately, there is very limited information regarding maturation of changes in these pacemaker structures and their influence on formation of the respiratory pattern during early maturation.
Neuronal populations within the nucleus of the solitary tract (NTS), retrotrapezoid nucleus (RTN), and medullary raphé nuclei integrate state-characterizing sensory information and influence the respiratory motor output in response to physiologic stimuli. The NTS is the site of termination of sensory afferents (slowly adapting stretch receptors (SARs), rapidly adapting stretch receptors (RARs), and C-fibers) from the lungs and airways. The NTS serves as the brain’s first processing center for sensory input from peripheral arterial chemoreceptors (3). Outputs from the NTS project to other respiratory nuclei and spinal phrenic motor neurons, informing the motor output pattern based on the status of the lungs and airways.
Maturation of Central CO2/H+ Chemosensitivity. Chemosensitive neuronal populations have been identified in a number of brain stem regions (5). The greatest density of CO2/H+ sensitive neurons is in the medullary raphé, ventral medullary surface, and retrotrapezoid nucleus (note that the rat retrotrapezoid nucleus (RTN) is believed to be homologous to the human arcuate nucleus) (6). Many chemosensory signals, transducers, and molecular mechanisms are involved in the process of chemoreception, the complexities of which are only beginning to be elucidated.
Ventilatory responses to CO2 are present at birth in most mammalian species, including humans. Even prenatally, exposure to CO2 incites increased depth of fetal breathing movements (7) (see later). In premature infants, CO2 sensitivity and the hypercapnic ventilatory response are diminished in the early postnatal period (8–10). Increased minute ventilation in response to CO2 in premature infants is primarily mediated by increases in tidal volume rather than respiratory rate (9). The hypercapnic ventilatory response in these infants is often associated with prolonged expiration characterized by expiratory braking and, in some infants, audible grunting (11). This may serve to preserve FRC. In contrast, older infants and adults, when exposed to CO2, have increased respiratory frequency characterized by a shortened expiratory phase. Further, infants with apnea of prematurity have reduced hypercapnic ventilatory responses relative to control infants at the same postconceptional age, suggesting that infants with apnea of prematurity have diminished central respiratory drive (12).
Another curious feature of the premature infant is the relationship between eupneic PaCO2 levels and the threshold below which decreasing PaCO2 will result in apnea, the so-called apneic threshold. In premature infants, the apneic threshold is much closer to eupneic levels of PaCO2 compared to adults (13) (Figure 12-3). The proximity between eupneic CO2 and the apneic threshold, coupled with baseline respiratory instability (affected by significant peripheral chemoreceptor drive to breathe in the neonatal period, low FRC, and sleep state) results in fluctuations in PaCO2 that are more likely to cross the apneic threshold in preterm infants, thus contributing to apnea of prematurity.
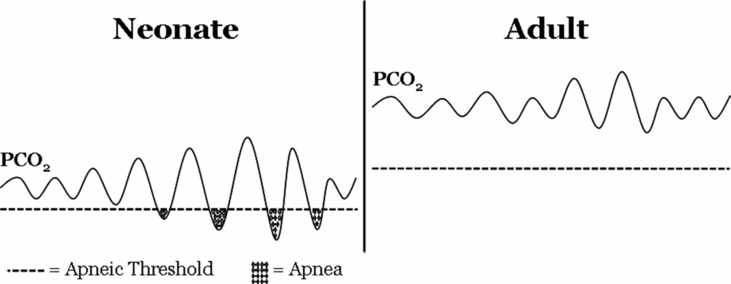
Relationship between eupneic PCO2 and apneic threshold in neonates and adults. Relative to adults, PCO2 during eupneic breathing is lower in the neonate and closer to the apneic threshold (dashed line). Neonates also have more variability in breathing, causing wider fluctuation in baseline PCO2. Together, these factors contribute to more frequent decreases in PCO2 below the apneic threshold, leading to cessation of breathing.
Mechanisms accounting for the postnatal maturation of central CO2 chemosensitivity are unknown. Improved chemoreception may relate to maturation of intrinsic properties of chemosensory neurons themselves or of their synaptic outputs. Alternatively, improved chemosensitivity may relate to changes in the relative importance of different central and peripheral chemosensory sites during development (14).
An important candidate gene presumed to regulate central and peripheral chemoreceptor development is PHOX2B, a homeobox gene located on chromosome 4 (15). Its expression is necessary for normal development of the carotid body and NTS. It is also expressed in chemosensitive glutamatergic neurons of the RTN. Mutations in the PHOX2B gene lead to congenital hypoventilation syndrome (CCHS), characterized by impaired hypoxic and hypercapnic ventilatory responses and apnea, especially during sleep.
Neurochemistry of Respiratory Control. Excitatory and inhibitory neurotransmitters mediate the rhythmogenic synaptic communications between neurons of the medullary CPG network. In addition, myriad neuromodulators, although not essential for rhythmogenesis, exert profound effects on respiratory rhythm and inspiratory and expiratory patterns (Figure 12-4). Neuromodulators are substances released by axon terminals within the respiratory network but whose cell bodies are outside the CPG. They include biogenic amines, acetylcholine and other neuropeptides, and defects in their function underlie a number of disease states.
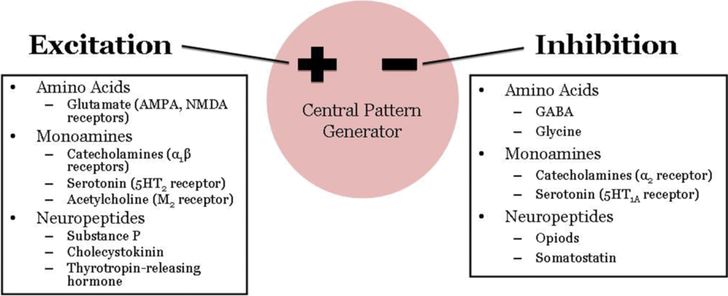
Excitatory and inhibitory regulators of respiratory neural output. Effects are dependent on the type of receptor activated and where within the network the mediators are acting.
Glutamate, acting on AMPA and NMDA receptors, is the major neurotransmitter mediating excitatory synaptic input to brain stem respiratory neurons and respiratory premotor and motor neurons. Within the CPG, glutamate is involved in generation of augmenting respiratory neuron activity and inspiratory termination (2). Gamma-aminobutyric acid (GABA) and glycine are the two primary inhibitory neurotransmitters in the network, mediating the waves of inhibitory postsynaptic potentials during the silent phase of respiratory neurons. GABAA receptors and glycine receptors activate ligand-gated chloride channels, resulting in membrane hyperpolarization and fast synaptic inhibition (16). GABA can also mediate slow synaptic transmission via GABAB receptors, which are G-protein coupled receptors. Interestingly, during late embryonic and postnatal development, GABA and glycine can mediate excitatory neurotransmission secondary to changes in the chloride gradient across the membrane. It is unclear how this phenomenon relates to the greater inhibition of respiratory output that characterizes early neonatal life. GABA-mediated inhibitory neurotransmission may reduce CO2 chemosensitivity exhibited by premature infants. In a rodent model, hypercapnia activates GABAergic neurons and GABAA receptor blockade eliminates the hypercapnia-induced prolongation of expiratory time in young animals (16).
The ventilatory effects of neuromodulators depend on the type of receptor activated and on the respiratory mechanism controlled by that type of receptor. For example, a neuromodulator may stimulate ventilation when acting in one area of the CPG and depress ventilation when acting elsewhere. Serotonin is of particular importance in the modulation of respiratory function. Serotonergic neurons are located in medullary raphé nuclei and project to the NTS, VRC, RTN, and phrenic motor nuclei. Serotonergic projections may represent the neuroanatomic substrate for the integration of cardiorespiratory responses (2). Defects in the medullary serotonergic system likely contribute importantly to the pathogenesis of sudden infant death syndrome (SIDS) and in the ventilatory instability observed in Rett syndrome (5,17).
Catecholamines (epinephrine, norepinephrine, and dopamine) elicit varied ventilatory responses depending on the type of receptor activated and the respiratory mechanism involved. Dopamine tends to act peripherally in the carotid bodies, while epinephrine and norepinephrine exert a tonic influence on central respiratory neurons (2). Acetylcholine acting on muscarinic receptors is likely involved in central chemoreception and the ventilatory response to CO2, while nicotinic receptors are involved at the final stage of respiratory output in effecting acetylcholine transmission at neuromuscular junctions. Other neuroactive peptides are often colocalized with excitatory and inhibitory neurotransmitters and act as auxiliary messengers. For example, opioids and somatostatin depress respiratory neuronal firing, while substance P, cholecystokinin, and thyrotropin-releasing hormone exert excitatory drive. The interactions between classical neurotransmitters and neuromodulatory peptides acting on different receptor types and in different locations within the respiratory network creates nearly endless possibilities for precise shaping and fine-tuning of respiratory output during development.
Peripheral Afferents and Carotid Body Chemosensitivity
The output controlling breathing from the central nervous system is synthesized by end organs, which, in turn, provide feedback via central pathways of pulmonary and lower airway vagal afferents. The sensory receptors of the lung with afferent fibers coursing in the vagus nerves are separated into three groups: SARs, RARs, and bronchopulmonary C-fibers. SARs and RARs innervate the pulmonary and airway mechanoreceptors via fast-conducting, myelinated fibers, whereas bronchopulmonary C-fibers have slowly conducting axons that lack myelination. These axons terminate in the NTS within the central nervous system. Second-order neurons from the NTS then send projections to phrenic motor neurons in the medulla, pons, and spinal cord. The selective activation and inhibition of SARs, RARs, and C-fibers each affect cardiopulmonary reflexes, collectively influencing the control of breathing.
Slowly Adapting Receptors (SARs). SARs are activated by lung volume and stretch to enhance of inspiratory effort, bronchodilation, and tachycardia (18). They project to ipsilateral subnuclei within the NTS at the rostrocaudal level of the area postrema. Second-order neurons are activated by SAR afferents, which synapse on pump cells (P-cells) and inspiratory-β (Iβ) cells. P-cells have phasic activity that tracks lung volume changes and are controlled by glycinergic inhibition during early inspiration and glutamatergic excitation during late inspiration and early expiration. When stimulated, P-cells induce changes that mimic the Breuer-Hering (B-H) reflex, whereas inhibition mimics environments with reductions in SAR input. Iβ cells are inspiratory neurons that, like P-cells, are monosynaptically excited by SARs. They receive input from both the ipsilateral and contralateral vagus nerve and show a ramp-like increase in firing during inspiration while being silent during expiration. These second-order neurons then send projections to the ipsilateral ventral respiratory column (VRC) that are involved in rhythmogenesis.
The reflexes controlled by pulmonary vagal afferents and SARs in humans are similar to other mammals. One example is the Breuer-Hering reflex, which controls the duration of inspiration and expiration in relation to lung inflation. Josef Breuer first showed in adult cats that expansion of the lungs reflexively inhibits inspiration and promotes expiration and deflation of the lungs (19). In this way, the B-H reflex protects against lung overinflation. This reflex is mediated by SARs that act through the NTS, P-cells, and Iβ cells as described earlier. The B-H reflex does not, however, participate in the regulation of fetal breathing movements because vagotomy in fetal sheep has little effect on the incidence, frequency, or amplitude of these movements (20). However, the B-H reflex is essential in establishing continuous breathing and adequate gas exchange at birth by maintaining functional residual capacity; vagotomy at birth results in respiratory failure and atelectasis (21–22).
In humans, the B-H reflex is elicited by occluding the airway at one of two times in the respiratory cycle: end expiration where the next occluded inspiratory effort is prolonged and expiratory effort shortened, or end inspiration, where the next occluded expiratory effort is prolonged and inspiratory effort is shortened. The inspiratory/expiratory effort after the occlusion maneuver is compared to the nonoccluded breath to calculate a percent change in inspiratory/expiratory time. Using this methodology, the B-H reflex was shown to contribute significantly to tidal breathing in newborns, with decreasing contributions throughout the first year of life (23). The lower lung volumes and higher chest wall compliance in preterm infants and newborns activate the B-H reflex, shortening expiratory time and prolonging inspiratory time. The B-H reflex is also enhanced by premature birth (24), prone sleeping position (25), active sleep (26), and respiratory distress syndrome. The immediate prolongation of expiration and resultant slowing of respiratory rate with CPAP is one presumed manifestation of the B-H reflex.
Rapidly Adapting Receptors. RARs are activated in response to lung deflation, mechanical stimulation, and inhaled irritants and stimulate cough, bronchoconstriction, laryngoconstriction, and airway mucous secretion (18). In contrast to SAR primary afferent fibers, individual RARs project centrally to multiple sites within the NTS, suggesting that each afferent contributes to multiple effects attributed to these receptors. Each RAR synapses on caudal levels of the ipsilateral NTS subnuclei with smaller projections on the contralateral NTS. Excitatory input from RARs is mediated by non-NMDA glutamate receptors while RARs do not express mRNA for GABA or glycine, making it unlikely they have substantial inhibitory activity. When RAR cells receive excitatory input from stimuli such as ammonia, they send projections to second-order inspiratory (Iγ) neurons in the NTS and bulbospinal neurons, which stimulate lung inflation (18).
Developmentally, RARs are important for restoring lung inflation in premature and term newborns. They are activated by the low lung volume and tidal breathing environments that are common in newborns. When activated, RARs activate augmented (sigh) breaths, which restore lung volume. The frequency of augmented breaths increases with decreasing gestational and chronologic age, and their description differs between newborns and adults (27). Newborns take two large inspiratory breaths in succession compared to one inspiratory effort in adults. Premature and newborn infants also can hypoventilate after an augmented breath, whereas adults will increase minute ventilation, suggesting that peripheral arterial chemoreceptor inputs may play a larger role in newborns. PaO2 rapidly increases, and PaCO2 rapidly decreases during an augmented breath, thereby reducing excitatory input from peripheral arterial chemoreceptors, decreasing respiratory drive, and resulting in apnea. Peripheral arterial chemoreceptors also play a role in initiating augmented breaths as denervation of the carotid sinus decreases the frequency of these maneuvers (28). Therefore, increased RAR activity during lung deflation, coupled with increased sensitivity of peripheral arterial chemoreceptors, contribute to the increased frequency of augmented breaths along with subsequent hypoventilation in premature infants.
C-Fiber Receptors. Bronchopulmonary C-fibers are unmyelinated vagal afferents that are activated by a variety of physical, environmental, and chemical stimuli, mainly capsaicin, carbon dioxide, lung edema, and elevated temperature. These neurons control rapid shallow breathing, cough, apnea, bronchoconstriction, laryngoconstriction, airway mucous secretion, vasodilation, and bradycardia (18). They terminate mainly in the ipsilateral NTS with small projections sent to the contralateral side. Excitatory input is mediated by glutamate acting on non-NMDA receptors in NTS subnuclei. When stimulated, C-fibers release neuropeptides, mainly substance P, which have local direct effects such as bronchoconstriction, increased mucous secretion, and bronchial and nasal vasodilation. The central effects of C-fibers are mediated by second-order interneurons in the central nervous system that have not been identified to date. The end result, however, is reflex apnea characterized by prolonged expiratory time from excitation of these postinspiratory neurons and continuous firing of central expiratory neurons.
In newborns, stimulation of pulmonary C-fibers causes bronchoconstriction and apnea (29). Capsaicin-induced apnea was most sensitive in newborn rat pups younger than 10 days of age (30). Other stimuli such as acidosis, adenosine, reactive oxygen species, hyperosmotic solutions, and lung edema also stimulate C-fibers and enhance their effects. C-fibers can also be sensitized by inflammatory mediators in the local environment, which may play a role in the apnea typically observed in infants with viral infections such as respiratory syncytial virus (31).
Maturation of Peripheral CO2/H+ and Hypoxic Carotid Body Chemosensitivity. The carotid body is primarily responsible for the control of ventilation in response to arterial oxygen tension. Several histological cell types and their corresponding functions have been identified within the carotid body. Type I, or glomus, cells are the oxygen-sensing cells. Exposure to acute hypoxia results in glomus cell depolarization and neurotransmitter release through voltage-gated calcium channels. Type II cells, similar to glial cells, are not chemosensitive. Postsynaptic afferent nerve fibers oppose the glomus cells and have cell bodies in the petrosal ganglion. The primary target for afferents from peripheral arterial chemoreceptors is the commissural nucleus of the NTS. Glutamate binds to both NMDA and non-NMDA receptors on second-order neurons in the NTS and is responsible for transmission of excitatory inputs from the peripheral arterial chemoreceptors (32). These second-order neurons then send tonic excitatory projections to the RTN, the dorsal respiratory group, and the ventral respiratory group.
The contribution of the peripheral arterial chemoreceptors has been elucidated by separately perfusing the carotid and systemic circulation or measuring direct carotid body output. These studies have determined that peripheral chemoreceptors respond more rapidly to CO2/H+ than central chemoreceptors (33). These chemoreceptors do not appear to influence fetal breathing, but they are necessary for stabilization of breathing patterns because denervation of the carotid body in newborn animals will result in apnea and death (34). The maturation of the carotid body parallels the increased sensitivity of the glomus cell to excitatory and inhibitory inputs. Newborn animals have increased sensitivity to CO2 during development, although the contribution of peripheral versus central CO2 chemosensitivity to this maturational change is unclear (35).
There is a critical period in the first two weeks of postnatal development during which exposure to chronic hypoxia, chronic hyperoxia, and intermittent hypoxia can lead to persistent alterations of chemoreceptor function in animal models (36–37). Similar exposures outside these periods have little to no lasting effect, indicating a level of plasticity in the control of the respiratory system. It remains unclear whether this phenomenon has clinical consequences in humans. At birth, there is an acute insensitivity to changes in oxygen tension that gradually decreases during the first two to three weeks of postnatal development as hypoxic excitation and hyperoxic inhibition of breathing become stronger (38). The current speculation is that the sensitivity of peripheral chemoreceptors to hypoxia is “reset” at birth during the transition from fetal hypoxic to neonatal normoxic life. Exposure to hyperoxia or hypoxia from birth delays the maturation of the oxygen chemoreceptor response in animal models (36). A premature infant is born into a relatively hyperoxic environment, often exacerbated by iatrogenic hyperoxia from respiratory support. Interestingly, this chemoreceptor-resetting phenomenon is also observed in preterm infants who demonstrate enhanced peripheral arterial chemoreceptor influences on breathing after the same critical two- to three-week period. This period coincides with the development of periodic breathing, which is associated with excessive peripheral chemoreceptor activity.
Peripheral arterial chemoreceptors in the carotid body are the primary sites for detection of changes in arterial oxygen tension. Near term, fetal chemoreceptor activity is generally absent and responds poorly to hypoxia. The response to a hypoxic exposure is to increase ventilatory drive and behavioral arousal, and this reflex strengthens with maturation. Exposure to hyperoxia (Dejours test) will reduce ventilation in both animals and humans. Hypoxia induces depolarization of the carotid body glomus cell, activating voltage-dependent calcium channels, which lead to neurotransmitter release. The magnitude of the calcium-dependent response seems to be critical for the maturation of intact hypoxic ventilatory responses. Studies have shown the calcium-dependent current in rats increases with postnatal age until P14, which is paralleled by development of mature carotid body responses to hypoxia or anoxia (37). Again, we must infer characteristics of the human carotid body chemoreceptors by observing hypoxic ventilatory responses. If human infants are repeatedly exposed to intermittent hypoxia in settings such as prematurity, apnea, and periodic breathing, they demonstrate a greater reduction in minute ventilation when exposed to hyperoxia. This suggests a greater influence of peripheral arterial chemoreceptors on normal breathing in this population.
A normal response to hypoxia in animals with functioning peripheral and central chemoreceptors is an increase in ventilation within thirty seconds of hypoxic gas exposure and decrease after two to three minutes. The trend back to ventilatory baseline is referred to as the hypoxic roll-off, hypoxic ventilatory decline, or hypoxic ventilatory depression. The hypoxic roll-off remains above baseline in mature models but can trend below baseline and result in apnea in newborns. The mechanisms that account for hypoxic roll-off are mediated in the pons and under control of neuromodulators such as norepinephrine, adenosine, GABA, serotonin, opioids, and platelet-derived growth factor. Adenosine has garnered particular attention because during hypoxia, brain adenosine levels can increase over twofold in fetal sheep (39). Blockade of adenosine receptors with caffeine and other methylxanthines can decrease the hypoxic ventilatory depression in newborn infants. This is a mainstay of neonatal therapy in apnea of prematurity (discussed later).
Fetal Breathing
Fetal breathing activity can be identified by the eleventh week of gestation in the human fetus with ultrasound. Although the placenta is the site of gas exchange in utero, fetal breathing movement is important in enhancement of lung growth and development, and decreased diaphragmatic activity is associated with pulmonary hypoplasia. Fetal breathing occurs phasically only during rapid eye movement (REM) sleep with total cessation of breathing during non-REM sleep, possibly secondary to descending inhibitory pontine input to the medullary rhythm-generating center. Various factors may contribute to the inhibition of fetal respiratory activity as summarized in Figure 12-5. Most prominent of these is probably respiratory depression induced by the hypoxic intrauterine environment to which the fetus is so well adjusted. Presumably an increase in fetal breathing movements in response to hypoxia would be counterproductive. In contrast, hypercapneic exposure does increase the incidence and depth of fetal breathing, although only in REM sleep. Ability to generate a ventilatory response to CO2, the main chemical stimulus to breathing, is clearly important for a successful fetal to neonatal transition.
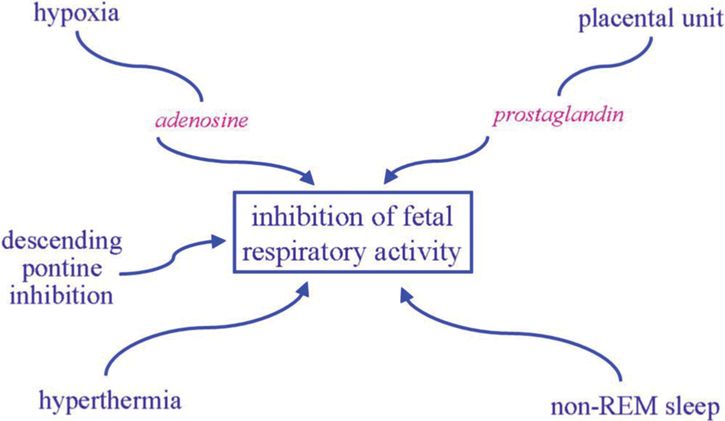
Factors contributing to the inhibition of fetal respiratory activity, notably the relatively hypoxic intrauterine environment.
Role of a Vulnerable Respiratory System
While immature respiratory control is the major contributor to apneic events in preterm infants, several components of the infants’ respiratory system increase vulnerability to cardiorespiratory events. Increased chest wall compliance and low lung volume both contribute to low oxygen reserves, often resulting in low baseline oxygen saturation (Figure 12-6). As a result, even short respiratory pauses may result in desaturation and resultant reflex bradycardia. This is consistent with the observation that episodes of intermittent hypoxia are significantly more frequent when baseline oxygen saturation is targeted at 85–89 versus 90–95% (40).
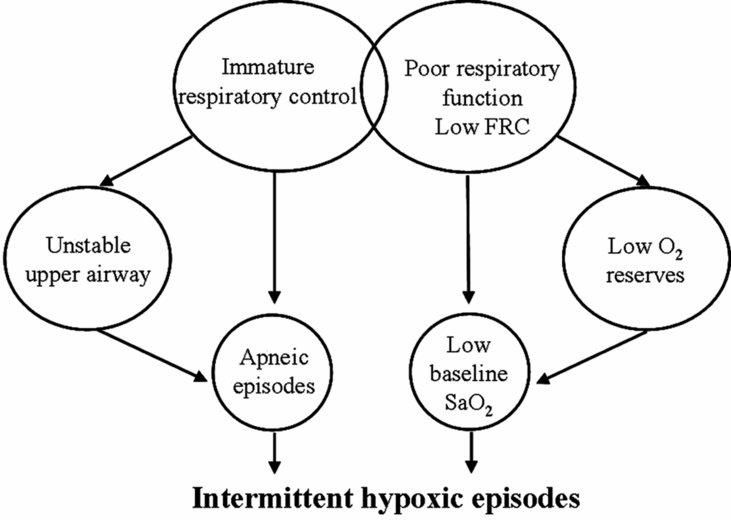
The net effect of immature respiratory control superimposed on a low lung volume and low intrapulmonary oxygen reserves is recurrent desaturation in the face of short respiratory pauses.
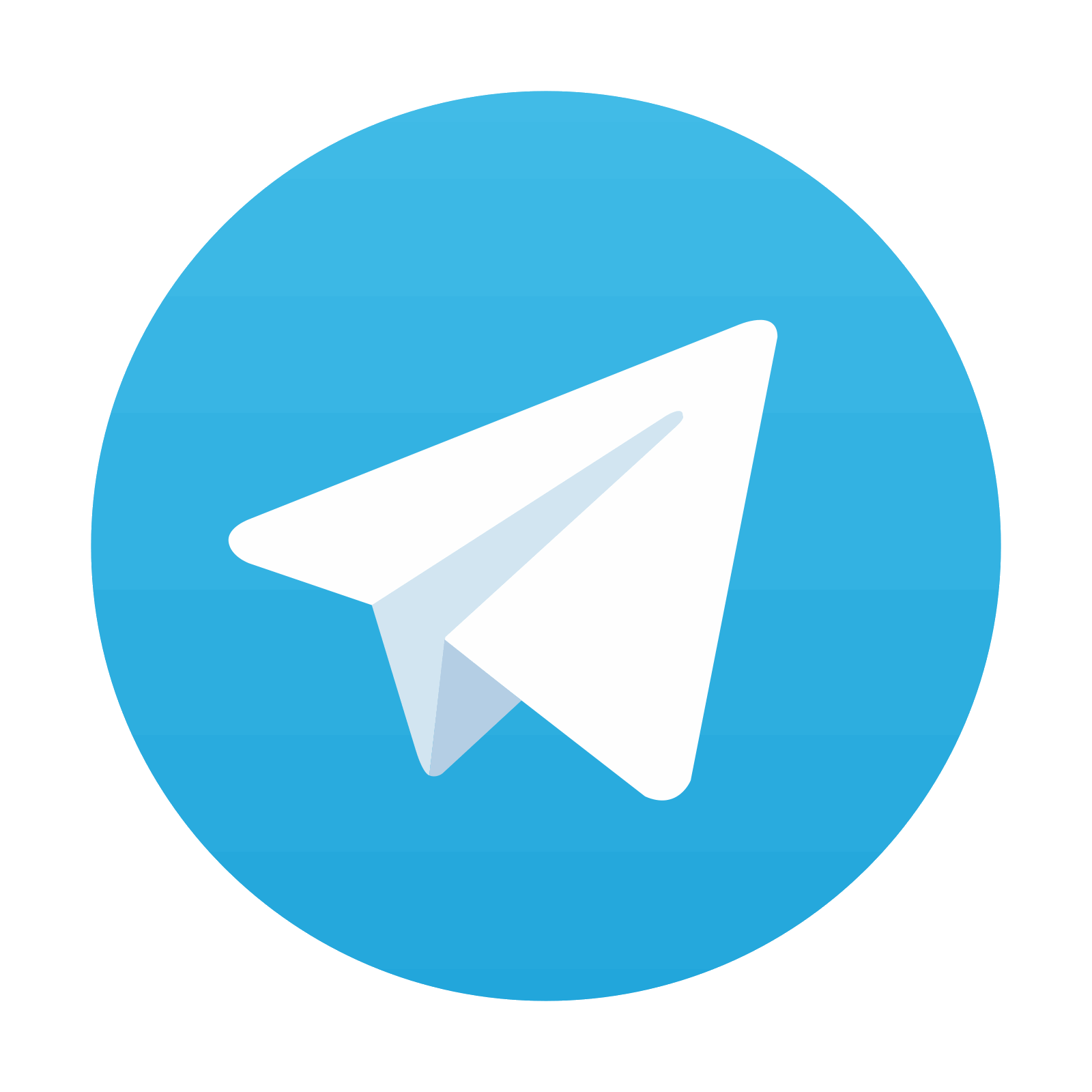
Stay updated, free articles. Join our Telegram channel
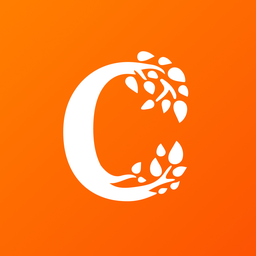
Full access? Get Clinical Tree
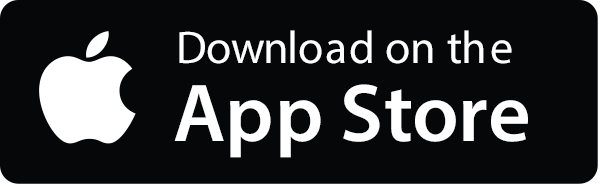
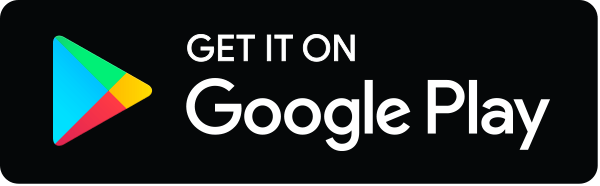