25
Work in hyperbaric environments
This chapter summarizes issues of both direct and indirect relevance to the respiratory tract that are encountered when exposed to environmental pressure raised above that found at sea level (normobaric). Raised (hyperbaric) environmental pressure is achieved either by diving in a fluid or by entering dry pressurized environments such as underground works and recompression chambers.
Humans have been exposed to elevated pressures for subsistence, work or pleasure ever since the first person dived underwater. Over many thousands of years breath-hold diving developed from a means to collect food for survival into profitable, if hazardous, industries which harvested food and other desirable natural items and salvaged lost valuables from shallow waters.
Man’s desire to go deeper for longer was realized in the sixteenth century through the use of diving bells. These upturned air-filled vessels could be lowered underwater and the diver could enter to take a breath of air without needing to return to the surface.
Various methods of delivering breathing gases to individual divers have since been developed and are broadly divided into those that are self-contained and those that are supplied via hoses. Each of these categories is further divided into equipment that recycles exhaled breath for re-inhalation and equipment that does not.
As early as the seventeenth century well-intentioned and innovative individuals built precursors to modern hyperbaric chambers. Patients sitting inside these vessels were exposed to minimally raised or lowered pressure in an attempt to treat a range of diseases.
In the nineteenth century, mining and building works proliferated and became increasingly ambitious. Ingress of water was a problem for underground works near to an expanse of water, and also for a caisson sitting on a sea or river bed. Increased pressure was used to minimize this problem. The miners, tunnelers and caisson workers who worked in these conditions were also subjected to elevated pressure, but without immersion. These techniques are still used today.
Working breath-hold divers exist to the present day. There is now also a welldeveloped competitive sport based on breath-hold diving, although increasingly elaborate equipment, from suits and fins to weights and buoyancy devices, is required to allow the boundaries to be pushed ever further. The current record for depth on a single breath-hold is in excess of 200 meters.
Most recreational divers breathe compressed air, via a demand valve, supplied from a cylinder worn by the diver in an assembly known as self-contained underwater breathing apparatus (SCUBA). The diver’s exhaled air is released into the surrounding water. Equipment using this principle is known as ‘open circuit’. See Figure 25.1. Other gases mixed with oxygen are used for breathing by commercial divers and by ‘technical divers’ amongst the recreational fraternity when they wish to dive deeper than the usual recreational range. It is not unusual for breathing gas to be delivered to commercial divers by hose from the surface or from a bell with the advantage of greater endurance and assured communication with the surface but the disadvantage of limited mobility.
Figure 25.1 Open-circuit SCUBA. 1, Compressed gas. 2, First stage reduces gas pressure to a few bars above ambient. 3, Second stage delivers gas to diver at ambient pressure. 4, Exhaled gas bubbles disperse in water
Another form of underwater breathing apparatus allows the diver to rebreathe exhaled gas once it is scrubbed clean of carbon dioxide and the oxygen is replaced. These ‘rebreathers’ minimize wastage of expired gas. The advantage of greater gas economy comes at the cost of greater complexity and a requirement for more extensive maintenance and training. As depth and ambient pressure increase, the inhaled gas is compressed more, so that a greater ‘surface equivalent’ volume of gas is wasted in each breath in an open-circuit system. Rebreathers avoid this depth-related increase in gas consumption. In ‘closed’ rebreathers no gas escapes from the system when at a steady depth. ‘Semi-closed’ sets produce a constant stream of excess gas, but in volumes that are generally much less than from an ‘open-circuit system’ (Figures 25.2–25.4).
Figure 25.2 Closed circuit oxygen rebreather. 1, Oxygen added to counterlung either manually or by a demand valve mechanism. 2, Relief valve to allow expanding gas to escape from counterlung on ascent. 3, Mouthpiece – one-way valves ensure that gas goes only in one direction in the circuit. 4, Exhaled gas passes through a carbon dioxide absorbent before returning to the counterlung
Figure 25.3 Semi-closed circuit rebreather. 1, Reducer delivers a constant mass of gas per unit time to the counterlung throughout dive and at all depths; proportion of oxygen is calculated to avoid hypoxia and hyperoxia between surface and maximum intended depth; rate is calculated to accommodate expected oxygen consumption; extra gas added either manually or automatically to make up for volume lost on descent. 2, Relief valve to allow gas to escape from counterlung 3, Mouthpiece – one-way valves ensure that gas goes only in one direction in the circuit. 4, Exhaled gas passes through a carbon dioxide absorbent before returning to the counterlung
Figure 25.4 Automated closed circuit mixed gas rebreather. 1, Sensors monitor oxygen levels in the circuit and oxygen or mixture is added to the counterlung to maintain oxygen at the desired level; nonautomated versions require the diver to add the required gas; extra gas added either manually or automatically to make up for volume lost on descent. 2, Relief valve to allow expanding gas to escape from counterlung on ascent. 3, Mouthpiece – one way valves ensure that gas goes only in one direction in the circuit. 4, Exhaled gas passes through a carbon dioxide absorbent before returning to the counterlung
For deep, long dives commercial divers can remain at pressure for weeks at a time, traveling between working depth and the surface in a pressurized diving bell. Shifts of some 6-8 hours are spent working in the water with rest periods in a dry, pressurized chamber facility on-board a diving support vessel. This is known as saturation diving (Figure 25.5).
Hyperbaric chambers are now used to treat decompression illness (DCI) and a range of other conditions which, for therapeutic effect, require inspired partial pressures of oxygen greater than those that can be achieved at sea level.
Commercial and recreational divers are exposed to both raised pressure and immersion. Tunnelers, caisson workers and hyperbaric chamber staff are, in general, exposed solely to raised pressure.
Surface-oriented working divers return to normobaric conditions after each dive. The depth and duration of the dive dictate the rate at which they may ascend. These divers are transported to and from their worksite in the water either by their own propulsion, by small powered vehicles, or lowered in a basket or in a bell which can be fully closed or partially enclosed. Tunnelers and hyperbaric chamber workers enter enclosed spaces which are compressed to allow them to work in a pressurized environment and then decompressed to allow them to return to normal atmospheric pressures.
In the UK the annual fatal accident rate for all diving at work activities is estimated at 6-7 per 100,000. There are approximately three cases of DCI each year in the offshore sector. This can be compared with the UK recreational sector in which, among perhaps 100,000 divers, there are approximately 16 fatalities and 200-250 cases of DCI per year and with compressed air work which is associated with some 25 cases of DCI (in approximately 2000 workers) per year.
An exposure is quantified primarily by the mixture(s) of gases breathed, the ambient pressure and duration for which each is breathed and whether the individual is immersed. Pressure is typically expressed as a depth in diving, increasing by approximately 100 kPa for each descent of 10 m (or 33 feet) in seawater. In compressed air work pressure is typically measured in bars of overpressure above atmospheric (1 bar is approximately 100 kPa). The permitted overpressure is usually limited to 3.5 bars in the UK.
There is a considerable amount of information beyond basic quantification of the exposure that can be collected to assist in diagnosis and management of pressure-related disorders. This information, and its significance, is summarized in Tables 25.1 and 25.2.
Table 25.1 Points to explore in the history of an individual with symptoms and signs attributed to exposure to raised environmental pressure – details regarding the exposure
Item | Significance |
Occupation/pastimes | Useful for identifying any relevant additional exposures, e.g. respiratory sensitizers |
Type of exposure | For example, surface-orientated, saturation, dry, immersed Immersion pulmonary edema, saltwater aspiration syndrome and near-drowning require immersion Immersion increases the risk of cerebral oxygen toxicity |
Equipment | For example, SCUBA, open-circuit or rebreather, semi-closed, fully-closed Resistive load or large dead space in any diving equipment and absorbent failure or one-way valve malfunction in a rebreather are potential causes of hypercapnia Elevated PICO2 increases risk of cerebral oxygen toxicity Potential for diver to consume oxygen faster than it is delivered in a semi-closed rebreather can cause dilution hypoxia or hypoxia of ascent Potential for exhaled inert gas to accumulate in a closed-circuit pure oxygen rebreather leading to risk of dilution hypoxia or hypoxia of ascent It is possible to rupture the respiratory tract with forceful exhalation against resistance or by overpressurizing the breathing circuit Hyperventilation before a breath-hold dive increases the risk of hypoxia |
Other environmental information | For example, temperature, visibility, current, surface conditions Immersion pulmonary edema typically occurs in divers in cold water or who have been working hard, such as swimming against a current, exercise, shivering |
Timing and nature of untoward incidents | This might not be an illness induced by immersion or pressure. Remember to ask about other potential exposures, e.g. breathing gas contaminants, dust, hydrocarbon fumes, welding fumes, underwater blast, and their relationship to the onset of symptoms Anxiety is a risk factor for cerebral oxygen toxicity |
Depths/durations/inspired gas mixtures | These data are required to calculate decompression obligation. Post-incident gas analysis data might be available CNS oxygen toxicity unlikely to occur in a dry chamber at PIO2 below 200 kPa CNS oxygen toxicity can occur if PIO2 exceeds approximately 140 kPa while immersed. A hyperoxic seizure can lead to drowning, near-drowning, aspiration or an uncontrolled ascent with closed glottis causing pulmonary barotraumas ±DCI Pulmonary oxygen toxicity can occur if PIO2 exceeds 50 kPa. Units of Pulmonary Toxic Dose can be calculated to assess potential Acute hypoxia can occur if PIO2 falls below 15 kPa A depressurization from a depth of 1 m or greater in water can cause rupture by pulmonary overinflation. A dive on air or another oxygen nitrogen mixture at a depth of 30 m or deeper increases risk of hypercapnia in a CO2 retainer |
Gas consumption | Voluntary hypoventilation in order to conserve open circuit gas supply is a risk factor for hypercapnia It is possible to rupture the respiratory tract with a maximal inhalation; this might be an important mechanism in the rupture of lungs in divers who ‘skip-breathe’, deliberately holding themselves in prolonged inspiration in an attempt to reduce gas consumption |
Rapid or uncontrolled ascents | Risk factor for pulmonary barotrauma and for DCI |
Omitted decompression stops | Risk factor for DCI |
Method used to calculate decompression | For example, decompression table, computer, instinct Allows potential for omitted decompression to be assessed |
Activities during dive | Hypercapnia typically occurs in divers who have been working hard at depth Working hard is a risk factor for cerebral oxygen toxicity |
Table 25.2 Points to explore in the history of an individual with symptoms and signs attributed to exposure to raised environmental pressure – post-exposure details
Item | Significance |
Flying or other altitude exposure after dive | Risk factor for DCI, impact decreases the longer the delay after diving. Recommended delay can be up to 48 hours depending on type of diving |
Activities after dive | Working hard after a dive increases the number of circulating bubbles and is believed to be a risk factor for DCI |
Timing, severity, nature, rate of onset and subsequent evolution of manifestations | DCI, pulmonary barotrauma of ascent and hypoxia of ascent can arise only once decompression has begun Signs and symptoms of pulmonary oxygen toxicity, DCI, pulmonary barotraumas, immersion pulmonary edema, saltwater aspiration syndrome and near-drowning persist for some time beyond acute provocative exposure. Signs and symptoms of hypoxia typically do not. The respiratory symptoms of hypercapnia will resolve rapidly on termination of the exposure but a throbbing headache typically persists DCI can occur during decompression in saturation diving. In most other circumstances 50% of DCI cases will manifest within 1 hour of surfacing and 90% within 6 hours The initial symptoms of arterial gas embolism typically present during the ascent or within the first 10 minutes after surfacing. Hemiplegia, hemiparesis and reduced level of consciousness commonly occur. It is not unusual for the condition to improve then relapse later In view of the indiscriminate nature of the bubbles, it is not unusual to have a number of manifestations, for them to appear at different times and to evolve in different ways |
Response to treatment | For example, normobaric oxygen, fluids, recompression Although normobaric oxygen is an important first aid measure it can mask symptoms and signs of DCI which then appear when the treatment is discontinued Remember that a response to recompression does not necessarily confirm a diagnosis of DCI and that a failure to respond does not necessarily exclude DCI |
Past medical history, including any previous diving illness | Remember that other disorders can present coincidentally Pyrexia is thought to be a risk factor for cerebral oxygen toxicity |
Exposures are typically recorded in the individual’s personal logbook and, in the case of commercial exposures, in the records kept by the employer. Each entry will usually describe pressure/depth, duration, environmental conditions, breathing equipment, gas(es) and decompression schedule used plus a note of any adverse incidents.
25.2 Respiratory hazards, diseases and their management
25.2.1 Acute pulmonary effects of immersion
Water is much denser than air. Differential pressures applied to the body are negligible in air. They become significant in water to the extent that effort of ventilation may be noticeably affected unless the mouth is at the same depth as the lungs. The pressure also neutralizes gravitational pooling of blood in dependent limbs, redistributing blood to the thorax, increasing right heart filling, inducing an immersion diuresis and reducing lung capacity. This redistribution is potentiated by cold so, while head-out immersion in thermoneutral water at 35°C reduces vital capacity by 5%, it will fall by 10% in water at 20°C.
25.2.2 Pulmonary oxygen toxicity
Atelectasis can occur when breathing a high fraction of oxygen in normobaric and hyperbaric conditions. Breathing oxygen at partial pressures greater than 50 kPa can cause additional pathological changes of inflammation spreading from the carina, suppression of surfactant production and eventual fibrosis of respiratory epithelium. The greater partial pressures of oxygen achievable in hyperbaric environments accelerate the pathological process. Symptoms and signs range from an isolated sensation of airway irritation through crackles and wheezes, fever, thick secretions and bronchial breathing, to respiratory failure due to impaired gas exchange across inflamed respiratory epithelium. Lung volumes, flows, compliance and gas transfer all deteriorate, and the earliest changes usually precede symptoms. Large, rapidly reversing flow changes are more likely to be due to bronchoconstriction mediated vagally following a direct toxic effect of oxygen on the central nervous system.
Management of pulmonary oxygen toxicity
Clinical findings and results of investigations will depend on the severity of the condition. Sometimes bilateral opacities are seen on chest X-ray. Treatment requires the inspired partial pressure of oxygen to be reduced to 50 kPa or lower. Most changes apart from fibrosis are reversible and symptoms will start to improve within 2 hours. They are expected to resolve within 3 days of cessation of exposure, although an upper respiratory tract infection soon after the exposure can provoke symptom recurrence. Lung function improves rapidly at first, but a complete return to normal can take several weeks.
It is possible to predict the likely pulmonary consequences of an oxygen exposure using the concept of units of pulmonary toxic dose (UPTD). The relationship between UPTDs and clinically measurable variables is non-linear (see Figures 25.6 and 25.7) and there is considerable inter-individual variation.
Figure 25.6 Units of pulmonary toxic dose accumulated per minute plotted against inspired partial pressure of oxygen
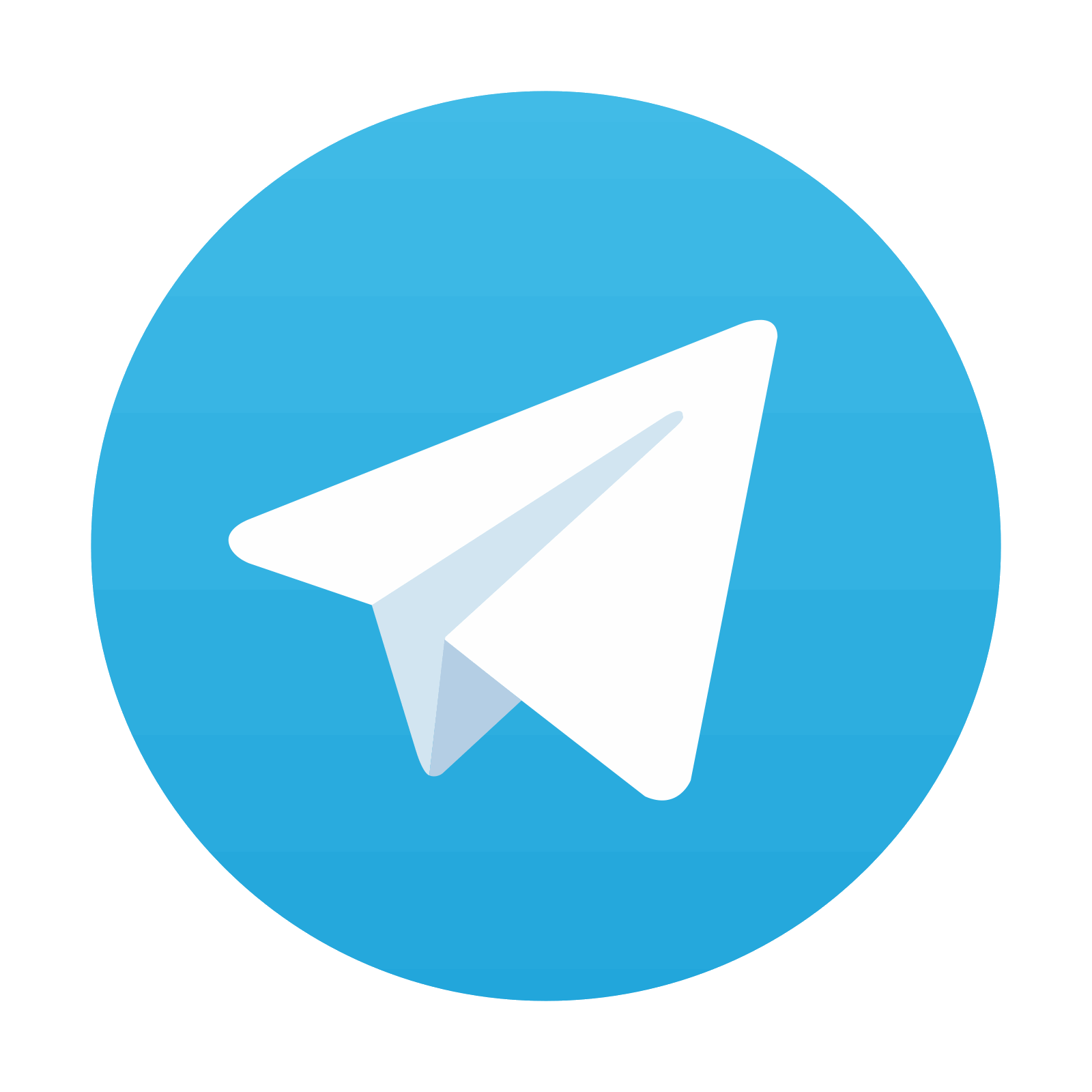
Stay updated, free articles. Join our Telegram channel
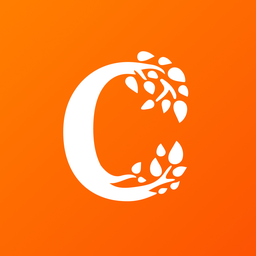
Full access? Get Clinical Tree
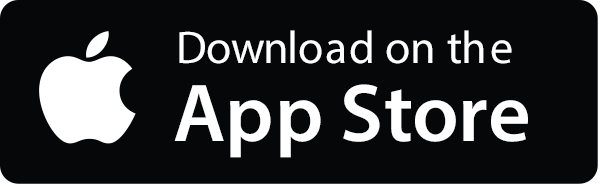
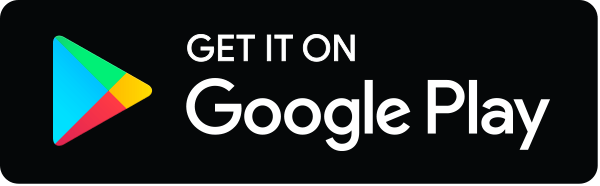