1. Severe symptoms of HF with dyspnea and/or fatigue at rest or with minimal exertion (NYHA functional class III or IV)
2. Episodes of fluid retention (pulmonary and/or systemic congestion, peripheral edema) and/or reduced cardiac output at rest (peripheral hypoperfusion)
3. Objective evidence of severe cardiac dysfunction, shown at least by one of the following:
(a) Low LVEF (<30%)
(b) Severe abnormality of cardiac function on Doppler echocardiography with a pseudonormal or restrictive mitral inflow pattern
(c) High LV filling pressures (mean PCWP >16 mmHg and/or mean RAP >12 mmHg by pulmonary artery catheterization)
(d) High BNP or NT-proBNP plasma levels, in the absence of non-cardiac causes
4. Severe impairment of functional capacity shown by one of the following:
(a) Inability to exercise
(b) 6-MWT distance <300 m or less in females and/or patients aged ≥75 years
(c) Peak VO2 < 12–14 mL/kg/min
5. History of ≥1 HF hospitalization in the past 6 months
6. Presence of all the previous features despite “attempts to optimize” therapy including diuretics, inhibitors of the renin-angiotensin-aldosterone system, and beta-blockers, unless these are poorly tolerated or contraindicated, and CRT, when indicated
Patients with advanced heart failure refractory to medical management may be eligible for advanced therapy, including heart transplantation and mechanical circulatory support (MCS) . Heart transplantation is considered the definitive therapy for advanced HF. However, shortage of donor organs and prolonged wait times remain a significant limitation. The development of MCS, such as the left ventricular assist devices (LVAD) , has emerged as an effective and viable form of therapy. Though this field is quickly evolving, therapy with an LVAD is not free of complications, making appropriate patient selection imperative for successful therapy.
Generally, LVAD implantation is considered reasonable in “highly selected patients with advanced end-stage HF and an estimated 1-year mortality >50% with medical therapy” [5].
Four major indications for LVAD implantation exist: (1) bridge to transplantation (BTT) , (2) destination therapy (DT) , (3) bridge to recovery, and (4) bridge to decision. Bridge to transplantation is considered in patients with advanced HF who are candidates for heart transplantation but are hemodynamically unstable despite maximum medical therapy, including inotropes and intra-aortic balloon pumps. Due to hemodynamic instability, prolonged wait time, and increased risk mortality, they are too ill to wait for a donor heart to be identified and require mechanical support in the interim. MCS in this population improves quality of life and reduces mortality [6]. In a multicentered trial, Frazier et al. demonstrated LVAD implantation improves NYHA functional class, organ dysfunction, and survival to transplantation compared to medical management in a cohort of transplant candidates. Furthermore, this survival benefit is sustained 1-year posttransplantation [7, 8]. Bridge to transplantation remains the most common indication for implantation [9]. However, due to the growing population of patients with advanced HF, scarcity of donor organs, and improved durability of the newer designed devices, there is an increasing trend toward LVAD implantation in patients for destination therapy. Destination therapy is offered as a permanent device in patients with advanced HF who are not candidates for heart transplantation. Like BTT, LVAD implantation as DT has also been shown to improve survival. The landmark Randomized Evaluation of Mechanical Assistance for the Treatment of Congestive Heart Failure (REMATCH) trial randomized 129 patients with end-stage HF who were not eligible for cardiac transplantation to LVAD therapy (HeartMate VE device) versus optimal medical management. LVAD therapy demonstrated a substantial survival benefit with 1-year survival of 52% versus 25% and 2-year survival of 23% versus 8%. Patients in the LVAD group also had improved quality of life, though they were two times more likely to develop serious adverse events including infection, bleeding, and mechanical failure of the device [10]. The development of newer left ventricular assist devices has further improved survival and quality of life. The HeartMate II trial randomized 200 patients with refractory HF who were ineligible for transplantation to the newer continuous-flow device versus pulsatile-flow device. Patients with the continuous-flow device demonstrated superior survival rates with 1-year survival of 68% versus 55% and 2-year survival of 58% versus 24%. While both groups had improvements in NYHA class and quality of life scores, the continuous-flow group had less major adverse effects [11]. Survival for continuous-flow pumps has continued to improve to an overall 1-year survival of 90% with 2-year survival of 70% [9, 12, 13]. Improved survival is not only secondary to advancements in LVAD technology but also to an improved understanding of heart failure, patient selection, surgical technique, and postoperative care [14]. Bridge to recovery is used in patients with potentially reversible etiologies of heart failure. LVAD implantation allows for recovery of myocardial function and is removed once the myocardium has recovered. Finally, bridge to decision is reserved for patients with hemodynamic instability requiring urgent mechanical support in which candidacy for transplantation or destination therapy cannot be made at the time of implantation. MCS at this time may allow for change in transplant eligibility with reversal of pulmonary hypertension, improvement in renal function and/or hepatic function, and weight loss.
General Criteria for MCS Patient Selection
Patients must undergo a comprehensive evaluation prior to LVAD implantations. The severity of heart failure must be assessed by the patient’s clinical presentation, cardiopulmonary stress testing, and hemodynamic studies. Cardiac and anatomic factors are also evaluated such as right heart function, presence of arrhythmias, and anatomic and body size. Non-cardiac factors including coexisting life-limiting illness, psychosocial, and age-related considerations are also assessed. Patients must also be evaluated for cardiac transplant candidacy and LVAD operative risk.
General indications for LVAD implantation are based on inclusion and exclusion data from clinical trials. LVADs are indicated in patients with severe HF with NYHA functional IV symptoms and left ventricular ejection fraction <25%, who have failed response to optimal medical management. This includes beta-blockers and ACE inhibitors if tolerated, inotropes, and intra-aortic balloon pumps. In those who are not inotrope- or balloon pump-dependent and physically able, functional limitation is demonstrated with peak oxygen consumption ≤14 mL/kg/min [15]. Absolute contraindications include sepsis or current active infections, severe right heart failure, untreated and severe carotid artery disease, severe obstructive and/or restrictive pulmonary disease, irreversible severe cerebral injury , dialysis-dependent renal failure, elevated INR from liver failure or disseminated intravascular coagulation, any severe end-organ failure, heart failure expected to recover without mechanical circulatory support, and non-cardiac illness that would limit survival to less than 2 years. Relative contraindications include morbid obesity, small body size (BSA <1.5 m2), chronic renal dysfunction but not dialysis-dependent, malnutrition, and severe or untreated mitral stenosis and aortic regurgitation. Of note, small body size may no longer be a contraindication as the newer devices are able to accommodate smaller body sizes [16].
Patient selection for LVAD remains a challenge as successful therapy is dependent on time of implantation and appropriate patient selection. The challenge lies with selecting patients with sufficient severity of illness to achieve a benefit while avoiding patients who are too ill or too early in the disease course to derive any benefit. Additionally, the risk of device-related complications including thromboembolic events, gastrointestinal bleeding, and infection must also be considered.
The National Institutes of Health-sponsored Interagency Registry for Mechanically Assisted Circulatory Support (INTERMACS) is a US registry which has acquired data on patients with FDA-approved MCS devices. It has developed a validated classification scheme based on patients’ hemodynamic status and has been established to predict outcomes in patients undergoing MCS. The classification scheme is felt to be more specific than NYHA classification and allows for optimal patient selection and timing. Moreover, it is easily assessed at the bedside (Table 2.2) [17].
Level | Hemodynamic status | Time frame for intervention |
---|---|---|
1. “Crash and burn” | Persistent hypotension despite rapidly escalating support and eventually IABP and critical organ hypoperfusion | Within hours |
2. “Sliding on inotropes” | Intravenous inotropic support with acceptable values of blood pressure and continuing deterioration in nutrition, renal function, or fluid retention | Within days |
3. “Dependent stability” | Stability reached with mild to moderate doses of inotropes but demonstrated failure to wean from them due to hypotension, worsening symptoms, or progressive renal dysfunction | Elective over weeks to months |
4. “Frequent flyer” | Possible weaning of inotropes but experiencing recurrent relapses, usually fluid retention | Elective over weeks to months |
5. “Housebound” | Severe limited tolerance for activity: comfortable at rest with some volume overload and often with some renal dysfunction | Variable urgency, dependent on nutrition and organ function |
6. “Walking wounded” | Less severe limited tolerance for activity and lack of volume overload. Fatigue easily | Variable urgency, dependent on nutrition and organ function |
7. “Placeholder” | Patient without current or recent unstable fluid balance. NYHA class II or III | Not currently indicated |
Currently LVADs are approved by the FDA for INTERMACS profile 1–5, with the greatest amount of implants in patients with profile 1–2 [9].
Risk Scores
No single variable exists to select candidates for LVAD therapy. While the INTERMACS classification scheme has been shown to predict prognosis after MCS, it lacks specificity and objectivity. Furthermore, it does not incorporate severity of multi-organ dysfunction [18]. Several risk scores have been developed, which incorporate individual data. They have been shown to predict both mortality in advanced heart failure and survival after LVAD therapy.
The Acute Physiology and Chronic Health Evaluation II (APACHE II) score was developed from a multi-institutional cohort of 5815 critically ill patients. The score consists of 13 variables including temperature, mean arterial pressure, heart rate, respiratory rate, partial pressure of arterial O2 or A-a gradient, arterial pH, sodium, potassium, creatinine, hematocrit, white blood cell count, Glasgow coma score, and age. Patients with HF and an APACHE II score greater than 20 have been shown to have a significant increase in hospital deaths [19]. In a retrospective cohort of patients with advanced HF, each unit increase in the APACHE II score independently predicted death. Furthermore, patients with a medium APACHE II score of 11–20 had the greatest benefit from LVAD placement [20].
The Seattle Heart Failure Model (SHFM) was derived from a cohort of 1125 NYHA IIIb–IV patients. Twenty variables are weighted by hazard ratio: age, gender, NYHA class, weight, ejection fraction, systolic blood pressure, presence of ischemic cardiomyopathy, daily furosemide equivalent dose, inotrope use, statin use, allopurinol use, angiotensin-converting enzyme/angiotensin receptor blocker use, beta-blocker use, potassium-sparing diuretic use, implantable cardioverter defibrillator, hemoglobin, lymphocyte percentage, serum uric acid, serum cholesterol, and serum sodium. The SHFM has been updated specifically for LVAD patients with the addition of intra-aortic balloon pump and inotrope therapy. Web-based calculators are available to convert the SHFM score to a mortality estimation where a score > 3.53 is considered high risk with a 50% predicted survival at 6 months. SHFM has been shown to predict 1-, 2-, and 3-year survival in low- to high-risk HF patients [21]. The performance of the SHFM was applied to advanced HF patients referred for transplant by Kalogeropoulos et al. Overall, the SHFM accurately discriminates between low- and high-risk patients. However, in terms of absolute risk (observed versus predicted event rate), the model overestimates survival and underestimates risk, particularly in black patients and those with implantable devices. The adverse events in this case were primarily driven by death. The model showed improved calibration when evaluating for mortality alone [22]. One limitation of the SHFM is that the model is not developed from a cohort of patients being considered for MCS. However, when applied to the REMATCH database, in which patients with advanced HF were ineligible for cardiac transplant, the 1-year SHFM-predicted survival was similar to actual survival for both the medical and LVAD therapy groups [23]. Furthermore, the SHFM is able to predict important features of the patient’s hospital course after LVAD implantation. Patients in the lower-risk group had shorter length of stay, higher rate of discharge, and discharge within 60 days of LVAD placement [24].
The REMATCH trial demonstrated that patients who were ineligible for cardiac transplant had improved 1-year survival with LVAD therapy compared to patients receiving optimal medical therapy. The Destination Therapy Risk Score (DTRS) was derived from the DT registry in the post-REMATCH era. In an analysis of 222 patients who received pulsatile LVAD, nine preoperative risk factors were identified to predict 90-day in-hospital mortality by multivariable analysis. This includes platelet count ≤148, serum albumin ≤3.3, INR >1.1, vasodilator therapy, mean pulmonary artery pressure ≤25, AST >45, hematocrit ≤34%, BUN >51, and no intravenous inotrope use. Each variable is assigned a weighted risk score, creating a cumulative score ranging from 0 to 27. Patients can be stratified to four risk categories based on probability of 90-day mortality. The score offers good discrimination between low- and high-risk groups with a 1-year survival of 69% versus 13%. Limitations of the DTRS include generalizability as the registry is composed from an older population and predominantly Caucasian males. Mechanical ventilation, intra-aortic balloon pump, and patient size were also not represented in the model due to small sample size. Lastly DTRS was not applied to newer generations of continuous-flow LVADs [25]. This was examined by Teuteberg et al. who applied the DTRS to 1124 patients with continuous-flow LVADs for BTT and DT. The DTRS in this lower-risk cohort demonstrated modest discriminatory capacity for 90-day in-house mortality. The score was able to discriminate between those at low versus high risk, however failed to discriminate between low- and intermediate-risk groups. The ability of DTRS to predict 2-year survival was also examined. DTRS moderately predicted survival for DT populations stratified by risk group, though it was unable to predict survival in the BTT population [26].
The HeartMate II risk score (HMRS) was developed from patients enrolled either in the HeartMate II (HMII) BTT or DT clinical trials. The score used preoperative patient-specific factors for predicting 90-day mortality in LVAD candidates. In a multivariate analysis, age (per 10 years), albumin, creatinine, INR, and center volume demonstrated good discrimination and calibration in predicting 90-day mortality. Patients were able to be stratified to three risk categories. When comparing HMRS to DTRS, HMRS provided significantly higher risk discrimination in both DT and BTT populations and across all risk groups [27]. However, this could not be confirmed in a single-center study by Thomas et al. In this retrospective analysis of 205 patients who received HMII for either BTT or DT, the HMRS demonstrated poor discrimination in 90-day and 1-year survival across all risk groups [28].
The above risk scores were applied to a cohort of 86 patients with continuous-flow LVADs to determine their ability to predict mortality after LVAD implantation. INTERMACS appeared to differentiate high-risk populations if patients with profile 1 and 2 were combined. The APACHE II and SHFM scores successfully differentiated between high-risk and low-risk groups. The DTRS failed to show significant survival between low-risk and high-risk groups. Overall, the SHFM score was the best predictor of mortality [29].
The development of risk scores based on preoperative risk is a useful guide to patient selection. However, no single risk score has been shown to be conclusively predictive and must be used in context with the patient’s clinical status.
Pulmonary Hypertension
Pulmonary hypertension is common in patients with advanced HF and is most commonly secondary to left heart disease. Pulmonary hypertension in this population is defined by mean pulmonary artery pressure ≥25 at rest and pulmonary arterial wedge pressure (PAWP) >15 mmHg assessed by right heart catheterization [30]. Pulmonary artery hypertension with elevated pulmonary vascular resistance (PVR) is considered a contraindication to heart transplantation when PVR >5 Wood units or the indexed pulmonary vascular resistance (PVRI) is >6 or the transpulmonary gradient exceeds 16–20 mmHg, due to the high risk of right ventricular failure posttransplantation [31]. This occurs because the grafted right heart is unable to tolerate an abrupt increase in pulmonary vascular resistance in the immediate postoperative period. The Cardiac Transplant Research Database has shown that preoperative vascular resistance is an independent risk factor for early and late mortality after cardiac transplantation [32].
While pulmonary hypertension is a contraindication for cardiac transplantation, it is not considered a contraindication for LVAD therapy. In fact, LVAD implantation may improve the severity of pulmonary hypertension , thereby reversing a patient’s contraindication to transplant candidacy. Numerous studies have demonstrated that LVAD implantation is safe and efficacious when used as bridge to transplant [33–35]. Tsuashita et al. conducted one of the largest studies evaluating the effect of continuous-flow LVADs on pulmonary hypertension as well as posttransplantation outcomes in patients bridged with LVADs. The study found that PVR decreased significantly post-LVAD implantation. This was also observed in patients considered to have severe refractory pulmonary hypertension. As a result, 66% of patients in this cohort, who would have otherwise not been candidates for transplantation, were able to have their eligibility reversed and undergo cardiac transplantation. Despite improvements in PVR post-implantation, patients with high PVR pre-LVAD implantation still had increased in-hospital mortality posttransplantation. A potential explanation for this finding is that the pulmonary vasculature undergoes heterogenous or incomplete remodeling and therefore is susceptible to early postoperative insult including myocardial ischemia, metabolic acidosis, hypoxemia, inflammatory response, or blood transfusion. Long-term survival posttransplantation , however, was similar in patients with high PVR pre-LVAD compared to those with low PVR [36].
Right Ventricular Failure
Right ventricular failure (RVF) after LVAD implantation is a cause of significant morbidity and mortality. It results in longer hospitalization, higher transfusion requirements, need for reoperation, and end-organ damage [37]. Concurrent placement of a right ventricular assist device (RVAD) for RVF has been identified as the most significant risk factor for death after implantation [38]. Several mechanisms contribute to RVF following LVAD implantation. Increased cardiac output from left ventricular unloading leads to increased venous return to the right ventricle, thereby unmasking preexisting right ventricular dysfunction. Left ventricular decompression may also result in leftward shift of the interventricular septum, decreasing septal contribution to right ventricle contraction [39].
The incidence of RVF ranges from 9.4 to 44%, depending on the definition of RVF [40]. The INTERMACS defines right heart failure as symptoms or findings of persistent right ventricular failure characterized by both of the following: (1) documentation of elevated central venous pressure (CVP) and (2) manifestation of elevated central venous pressure. Elevated CVP may be measured either directly (e.g., right heart catheterization) with CVP or right atrial pressure (RAP) >16 mmHg, findings of the significantly dilated inferior vena cava with the absence of inspiratory variation by echocardiography, or clinical findings of elevated jugular venous distension. Elevated CVP is manifested by clinical findings of peripheral edema, the presence of ascites or palpable hepatomegaly on physical examination or diagnostic imaging, or laboratory evidence of worsening hepatic or renal function. The severity of RVF may be further categorized as mild, moderate, severe, and severe-acute RVF (Table 2.3) [41].
Mild | – Post-implant inotropes, inhaled nitric oxide, or intravenous vasodilators not continued beyond post-op day 7 after VAD implant |
– No inotropes continued beyond post-op day 7 after VAD implant | |
Moderate | – Post-implant inotropes, inhaled nitric oxide, or intravenous vasodilators continued beyond post-op day 7 and up to post-op day 14 following VAD implant |
Severe | – Central venous pressure or right atrial pressure >16 mmHg |
– Prolonged post-implant inotropes, inhaled nitric oxide, or intravenous vasodilators continued beyond post-op day 14 following VAD implant | |
Severe-acute | – Central venous pressure or right atrial pressure >16 mmHg |
– Need for right ventricular assist device at any time following VAD implant | |
– Death during VAD implant hospitalization with RHF as the primary cause of death |
Predicting patients at risk of developing RVF post-LVAD implantation would improve patient selection. It would also allow clinicians to implement strategies to avoid RVF. Several studies have identified predictors of post-implantation RVF (Table 2.4) [42–47]. However, predicting RV response to LVAD implantation remains challenging as there is no consensus among these studies, and the data is limited by different definitions used by each trial to define RVF.
Table 2.4
Preoperative risk factors for RVF
Study (first author) | n | VAD type | Definition of RVF | RVF incidence | Preoperative risk factors |
---|---|---|---|---|---|
Ochai [42] | 245 | Pulsatile | – RVAD implantation | 9% | – Female gender (OR 4.5) |
– Preoperative circulatory support (OR 5.3) | |||||
– Nonischemic etiology (OR 3.3) | |||||
Dang [43] | 108 | Pulsatile | – RVAD implantation | 38.9% | – Female gender |
– Inotropes/pulmonary vasodilator therapy ≥14 days | – Elevated intraoperative CVP (OR 1.2) | ||||
Drakos [44] | 175 | Pulsatile (86%), Continuous (14%) | – RVAD implantation | 44% | – Destination therapy (OR 3.3) |
– Inotrope therapy >14 consecutive days | – IABP (or 3.9) | ||||
– Inhaled NO ≥48 h | – PVR ≥4.3 WR (or 4.1) | ||||
– PVR 2.8–4.2 Wu (or 3.0) | |||||
– Higher RAP | |||||
– Increased LV end-diastolic diameter | |||||
– Lower platelets | |||||
– Higher bilirubin | |||||
Fitzpatrick [45] | 266 | Pulsatile (98%), Continuous (2%) | – RVAD implantation | 37% | – Cardiac index <2.2 L/min/m2 (OR 5.7) |
– RVSWI <0.25 mmHg/L/m2 (OR 5.1) | |||||
– Severe pre-VAD RV dysfunction (OR 5.0) | |||||
– Cr ≥1.9 (OR 4.8) | |||||
– Previous cardiac surgery (OR 4.5) | |||||
– SBP ≤96 mmHg (OR 2.9) | |||||
Matthews [46] | 194 | Pulsatile (86%), Continuous (14%) | – RVAD implantation | 35% | – Vasopressor requirement (OR 3.9) |
– Inotrope therapy >14 days | – AST ≥80 (or 2.1) | ||||
– Inhaled NO ≥48 h | – Bilirubin ≤2 (OR 2.4) | ||||
– Hospital discharge with inotrope therapy | – Cr ≥2.3 (OR 2.9) | ||||
Kormos [47] | 484 | Continuous | – RVAD implantation | 20% | – CVP/PCWP >0.63 (or 2.3) |
– Inotrope therapy >14 days after implantation | – Preoperative ventricular support (OR 5.5) | ||||
– Inotrope support starting 14 days after implantation | – Bun >39 (or 2.1) |
Matthews et al. developed a RVF risk score (RVFRS) based on independent predictors of RVF. Each variable was weighted by odds ratio: vasopressor requirement (4 points), AST ≥80 (2 points), bilirubin ≥2 (2 points), and creatinine ≥3.0 (3 points). The RVFRS is calculated as the sum of the pointed awarded for the presence of each of the preoperative variables. Patients with a score ≥5.5 have a 15-fold greater risk of developing RVF compared to patients with a score ≤3.0. When compared to commonly used predictors of RVF-RVWSI, transpulmonary gradient, PVR, RAP, and PASP, RVFRS was shown to be superior [46].
Invasive hemodynamic monitoring also has a role in predicting RVF. Elevated CVP, low pulmonary artery systolic pressure (PASP) , low RV stroke work index, low cardiac index, and elevated PVR have all been shown to be predictors of RVF. Interestingly, unlike cardiac transplantation, low PASP rather than high PASP is a predictor of RVF. Caution must be used however as hemodynamic parameters may fluctuate in unstable patients who may be on inotropes .
A novel hemodynamic marker, the pulmonary artery pulsatility index (PAPi) , has been found to identify patients at high risk of developing severe RVF after inferior wall myocardial infarction [48]. PAPi is defined as [(systolic pulmonary artery pressure − diastolic pulmonary artery pressure)/central venous pressure]. When applied retrospectively to continuous-flow LVAD recipients, a higher PAPi (>2.0) was associated with lower rates of RVAD implantation. Interestingly, PAPi more strongly predicted early RVAD requirement when hemodynamics were measured on inotropes versus off inotropes. Furthermore, the predictive ability of PAPi remained valid regardless of the timing between right heart catheterization and LVAD implantation, where the maximum time was 6 months [49]. In another cohort of patients with continuous-flow LVADs, PAPi initially decreased in the immediate postoperative setting. However, in patients without RVF, PAPi significantly increased after 24 h. Therefore, PAPi may also be used as an indicator for right ventricular recovery following LVAD implantation [50].
Attempts have also been made to identify predictors of RVF by echocardiography. This is challenging due to the retrosternal position of the right ventricle and its complex geometry, as well as the lack of standardization of echocardiographic protocols [40]. Tricuspid annular plane systolic excursion (TAPSE) <7.5 mm [51], reduced right ventricular fractional change area (<35%) [52], short to long axis ratio ≥ 0.6, and more severe tricuspid regurgitation [53] have been found to be predictive of RVF in small, single-center studies. However, these parameters could not be validated in larger subsequent studies, potentially because measurements of these load-dependent values are made in patients under severe hemodynamic stress.
Strain, strain rate, and speckle tracking are promising predictors of RVF. Unlike standard echocardiographic predictors, strain imaging is less sensitive to loading conditions and can reasonably assess right ventricular systolic function. Strain imaging was applied to patients undergoing LVAD implantation. Global longitudinal right ventricular strain was found to be an independent predictor of RVF where a right ventricular free wall strain <−9.6% predicted RVF with 68% sensitivity and 76% specificity. When combined with the RVF score described above, it provided incremental value on its predictive ability [54]. The right ventricular function after left ventricular assist device (RFV-LVAD) study prospectively evaluated LVAD candidates with standard echocardiographic parameters and strain imaging. Standard echocardiographic parameters included TAPSE, pulse tissue Doppler peak systolic velocity, right ventricular myocardial performance index, and right ventricular fractional area change. Preliminary findings revealed right ventricular global longitudinal strain was the most important predictor of RVF [55].
With available predictive risk factors for RVF, the goal for the clinician should be to optimize the patient prior to LVAD implantation as well as certain measures during implantation and postoperatively [56, 57]. In the preoperative stage, patients with evidence of right ventricular dysfunction should undergo aggressive treatment to reduce right ventricular wall stress with a goal of decreasing right atrial pressure to <12 mmHg. The benefit of reducing pulmonary artery pressure and pulmonary vascular resistance remains uncertain as they have been shown to be inconsistent predictors of RVF. Though they may be decreased with PDE5 inhibitors, this has yet to demonstrate a clear clinical benefit. Those who remain at high risk despite medical optimization should be considered for planned biventricular support or total artificial heart as elective right VAD implantation has been shown to have better long-term survival versus emergency implantation [58]. Intraoperatively, correction of tricuspid regurgitation has been theorized to improve right ventricular function, though this remains controversial. In a review of 2000 patients who underwent LVAD implantation, concomitant correction of moderate-to-severe tricuspid regurgitation did not reduce early death or right VAD requirement. In fact, this was associated with worse early postoperative outcomes including renal failure, dialysis, reoperation, transfusion requirement, and greater length of stay [59]. Surgical hemostasis during LVAD implantation is critical to minimize blood product transfusion, thereby preventing volume overload of the right ventricle [57]. Inotropic agents in the immediate postoperative period are essential due to increased preload to the right ventricle. Pulmonary vasodilators may also be employed to decrease right ventricular afterload. Optimization of pump speed also avoids excessive leftward shift of the septum, thereby decreasing venous return. If the right ventricle fails despite these measures and is unable to maintain adequate LVAD flow, implantation of a right VAD is necessary.
Renal and Hepatic Dysfunction
Renal dysfunction is common in patients hospitalized for heart failure. Acute kidney injury (AKI) , defined as an increase in creatinine >0.3 mg/dL, is observed in 50% of patients admitted for acute decompensated heart failure and 70% of patients with cardiogenic shock [60]. AKI is an independent risk factor for all-cause mortality, cardiac-specific mortality, pump failure death, and increased hospitalization in patients with heart failure [61, 62]. The etiology of renal dysfunction in patients with heart failure is multifactorial due to intrinsic disease from chronic comorbidities and cardiorenal syndrome. In cardiorenal syndrome, poor cardiac output results in poor renal perfusion. Venous congestion is also thought to play a role via activation of the renin-angiotensin system and renal arterial vasoconstriction to maintain glomerular filtration rate (GFR) . The autoregulatory capability cannot be sustained and GFR ultimately declines. Hemodynamic changes also result in decreased GFR secondary to inflammation, endothelial dysfunction, and anemia [63].
Not surprisingly, preoperative renal dysfunction is associated with complications and mortality post-LVAD implantation. While renal dysfunction is a relative contraindication for LVAD and dialysis dependence remains an absolute contraindication, renal function has been shown to improve after LVAD implantation. This is due to improved renal perfusion and correction of the neurohormonal dysregulation. Studies have shown patients who had recovery in GFR had a slightly increased survival compared to those who had no improvements in GFR . In fact, patients who had a recovery of GFR to that of >60 mL/min/1.73 m2 had comparable survival to patients with normal renal function post-implantation. Positive predictors of improved renal function include the absence of diabetes, lower cardiac index preimplantation, lower body mass index, and use of intra-aortic balloon pump. Negative predictors include older age and the use of angiotensin-converting enzyme inhibitors/angiotensin receptor blockers. These variables however have not been externally validated [64–66].
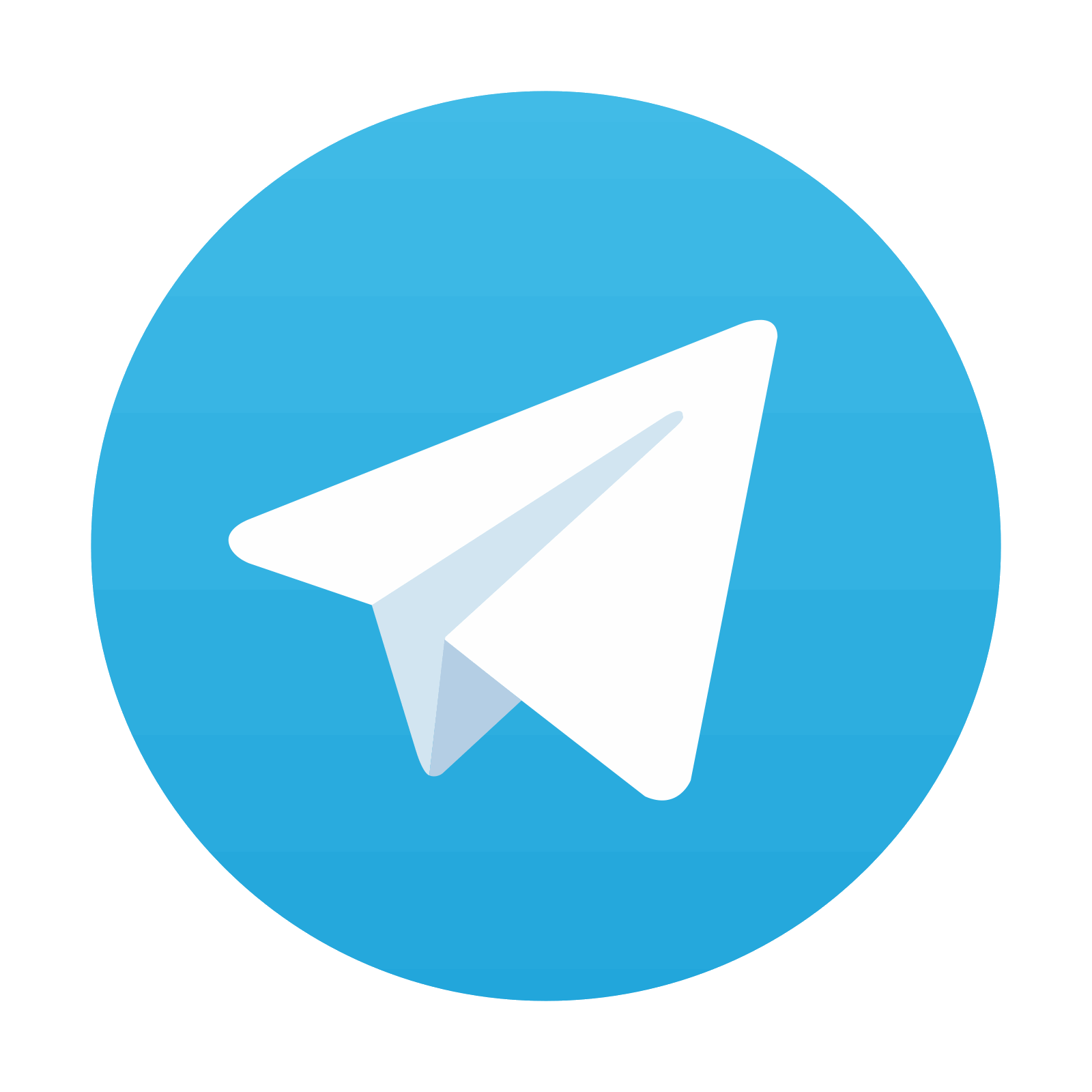
Stay updated, free articles. Join our Telegram channel
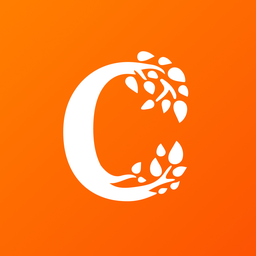
Full access? Get Clinical Tree
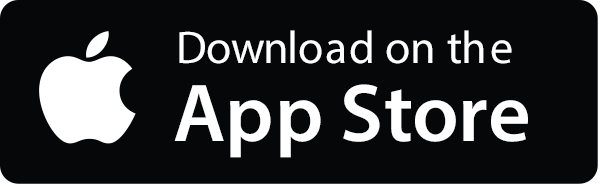
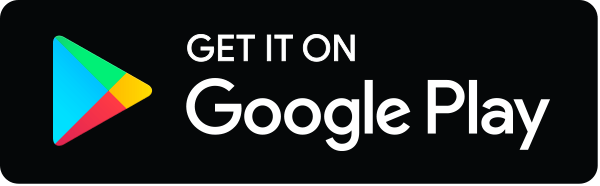