1 Voltage-gated sodium channels initiate action potentials in cardiac myocytes and other excitable cells, and they are responsible for propagation of action potentials through the atria, conduction system, and ventricles of the heart. As shown in Figure 1-1, action potentials in atrial and ventricular muscle fibers rise rapidly from a resting potential near −80 mV and reach their peak within 1 ms. During this brief interval, cardiac sodium channels respond to the change in pacemaker potential as it reaches threshold and open to allow rapid Na+ entry. Sodium channels begin to inactivate as soon as they open and inactivate to 98% or 99% completion within a few milliseconds. The plateau phase of the cardiac action potential is generated by opening of voltage-gated calcium channels (see Chapter 2), and the cell is finally repolarized by slower opening of voltage-gated potassium channels (see Chapter 3). The rate of conduction of the action potential through the cardiac tissue depends directly on the rate of rise of the cardiac action potential and therefore on the density of sodium channels and their rate of activation. Figure 1-1 Cardiac action potential in sheep heart. Cardiac myocytes in left ventricle or left atrium were impaled with a microelectrode, and the cardiac action potential was recorded. (Courtesy J. Jalife.) Sodium channel proteins purified from excitable cells are complexes composed of an approximately 260-kD α-subunit in association with one or two auxiliary β-subunits of approximately 33 to 39 kD.1 Purified sodium channel complexes of α- and β-subunits are sufficient for voltage-dependent gating and ion conduction in artificial lipid membranes and expression of the α-subunit alone is sufficient for physiologic function in recipient nonexcitable cells, indicating that this subunit has all the necessary structural elements for voltage-dependent gating and ion conduction.1–3 The primary sequence predicts that the sodium channel α-subunit folds into four internally repeated domains (I-IV), each of which contains six α-helical transmembrane segments (S1-S61,3–6; Figure 1-2). In each domain, the S1-S4 segments serve as the voltage-sensing module, and the S5 and S6 segments and the reentrant P loop between them serve as the pore-forming module. Extracellular loops connect the S5 and S6 transmembrane segments to the P loop in each domain, whereas the other extracellular loops are small. Large intracellular loops link the four homologous domains, and the large N-terminal and C-terminal domains also contribute substantially to the mass of the internal face of sodium channels. This view of sodium channel architecture, originally derived from hydrophobicity analysis of the amino acid sequence,4 has been largely confirmed by biochemical, electrophysiologic, and structural experiments.6 Figure 1-2 Transmembrane organization of sodium channel subunits. The primary structures of the subunits of the voltage-gated ion channels are illustrated as transmembrane folding diagrams. Cylinders represent probable alpha helical segments: blue, S1-S3; green, S4; yellow, S5; red, S6; shaded orange area, outer pore loop; purple, intracellular S4-S5 helix. Bold lines represent the polypeptide chains of each subunit with length approximately proportional to the number of amino acid residues in the brain sodium channel subtypes. The extracellular domains of the β1- and β2-subunits are shown as immunoglobulin-like folds Ψ, sites of probable N-linked glycosylation. P, sites of demonstrated protein phosphorylation by PKA (circles) and PKC (diamonds); white circles, the outer (EEDD) and inner (DEKA) rings of amino residues that form the ion selectivity filter and the tetrodotoxin binding site; ++, S4 voltage sensors; h in shaded circle, inactivation particle in the inactivation gate loop; open shaded circles, sites implicated in forming the inactivation gate receptor. The structure of the extracellular domain of the β-subunits is illustrated as an immunoglobulin-like fold based on amino acid sequence homology to the myelin P0 protein.8,22 Sites of binding of α- and β-scorpion toxins and a site of interaction between α- and β1-subunits are also shown. (Adapted from Catterall WA: From ionic currents to molecular mechanisms: the structure and function of voltage-gated sodium channels. Neuron 26:13–25, 2000.) The auxiliary β subunits were identified in the initial purification studies of sodium channels.1 These subunits have a single transmembrane segment, a large N-terminal extracellular domain that is homologous in structure to a variable chain (V-type) immunoglobulin-like fold, and a short C-terminal intracellular segment (see Figure 1-2).7–10 The β-subunits interact with α-subunits through their extracellular immunoglobulin (Ig)-fold domains, modulate α-subunit function, and enhance their cell surface expression.11 Like other proteins with an extracellular Ig-fold, they also serve as cell adhesion molecules by interacting with extracellular matrix proteins, cell adhesion molecules, and cytoskeletal linker proteins.12–17 These interactions are thought to localize and stabilize sodium channels in specific subcellular compartments and to bring crucial signaling molecules to the sodium channel to regulate it. Deletion of the genes encoding β-subunits causes alterations in sodium channel function; reduced action potential conduction and abnormal development of myelin folds in axons; hyperexcitability and epilepsy in the brain; and arrhythmias in the heart.18–20 Sodium channel architecture has been revealed in three dimensions by determination of the crystal structure of the bacterial sodium channel NavAb at high resolution (2.7 Å (0.27 nm); Figure 1-3). This ancient sodium channel has a simple structure—four identical subunits that are each similar to one homologous domain of a mammalian sodium channel without the large intracellular and extracellular loops of the mammalian protein.21 This structure has revealed a wealth of new information about the structural basis for sodium selectivity and conductance, the mechanism for block of the channel by therapeutically important drugs, and the mechanism of voltage-dependent gating. As viewed from the top, NavAb has a central pore surrounded by four pore-forming modules composed of S5 and S6 segments and the intervening pore loop (see Figure 1-3, A). Four voltage-sensing modules composed of S1-S4 segments are symmetrically positioned around the outer rim of the pore module (see Figure 1-3, A). The transmembrane architecture of NavAb shows that the adjacent subunits have swapped their functional domains such that each voltage-sensing module is most closely positional around with the pore-forming module of its neighbor (see Figure 1-3, B). It is likely that this domain-swapped arrangement enforces concerted gating of the four subunits or domains of sodium channels. Figure 1-3 Three-dimensional structure of sodium channels. A, Top view of NavAb channels colored according to crystallographic temperature factors of the main-chain (blue < 50 Å2 to red > 150 Å2). B, Side view of NavAb. C, Structural elements in NavAb. The structural components of one subunit are highlighted. 1-6, Transmembrane segments S1-S6. (Adapted from Payandeh J, Scheuer T, Zheng N, et al: The crystal structure of a voltage-gated sodium channel. Nature 475:353–358, 2011.) Comparison of the primary structures of the auxiliary β-subunits to those of other proteins revealed a close structural relationship to the family of proteins that contain Ig-like folds, which include many cell-adhesion molecules.8 The extracellular domains of these type-I single-membrane-spanning proteins are predicted to fold in a similar manner as myelin protein P0, whose Ig-like fold is known to be formed by a sandwich of two β-sheets held together by hydrophobic interactions (see Figure 1-222). Myelin P0 protein is a cell adhesion molecule involved in tight wrapping of myelin sheets, and many related cell adhesion molecules with extracellular Ig-folds and a single membrane-spanning segment are involved in cell-cell interactions among neurons and glia.22 As expected from their structure, NaVβ subunits interact with extracellular matrix molecules, other cell adhesion molecules, and intracellular cytoskeletal proteins and signaling proteins.12–16,23 Hodgkin and Huxley24 defined the three key functions of sodium channels: (1) voltage-dependent activation, (2) fast inactivation, and (3) selective ion conductance. Building on this foundation, detailed biophysical studies revealed the ion selectivity of the channel pore, detected the movement of the voltage sensors as gating current, and developed mechanistic models for these essential channel functions.25,26 Recent structure-function studies using molecular, biochemical, structural, and electrophysiologic techniques have provided clear understanding of the molecular and structural basis for these sodium channel functions. Voltage clamp studies showed that sodium channels are highly selective for sodium versus potassium and other monovalent cations.26,27 Analysis of ion selectivity and block by tetrodotoxin and saxitoxin led to a model of tetrodotoxin and saxitoxin as plugs of the selectivity filter in the outer pore of sodium channels.26 Mutational analysis identified a key glutamate residue in the membrane-reentrant loop in domain I as a crucial residue for tetrodotoxin and saxitoxin binding.28 Additional studies revealed a pair of important amino acid residues, mostly negatively charged, in analogous positions in all four domains (see Figure 1-2, B, small white circles).29–31 Mutation of a set of four residues in analogous positions in each domain (aspartate in domain I, glutamate in domain II, lysine in domain III, and alanine in domain IV, DEKA) to glutamates confers calcium selectivity,32 indicating that the side chains of these amino acid residues are likely to interact with sodium ions as they are conducted through the ion selectivity filter of the pore. These results showed that the narrow selectivity filter in the outer pore is formed by the reentrant P loops between transmembrane segments S5 and S6 of each domain (see Figure 1-2). Mutations in this postulated ring of four amino acid residues have strong effects on selectivity for organic and inorganic monovalent cations, in agreement with the idea that they form the selectivity filter, and structure-function studies suggest specific structural interactions and functional roles for the P loops from the four domains.33–36 In the bacterial sodium channel NavAb, the overall pore architecture includes a large external vestibule, a narrow ion selectivity filter containing the amino acid residues shown to determine ion selectivity in vertebrate sodium and calcium channels, a large central cavity that is lined by the S6 segments and is filled with water, and an intracellular activation gate formed at the crossing of the S6 segments at the intracellular surface of the membrane (Figure 1-4, A). The activation gate is tightly closed in the NavAb structure (see Figure 1-4, B), and there is no space for ions or water to move through it. This general architecture resembles voltage-gated potassium channels (see Chapter 3). Although the overall pore architecture of sodium and potassium channels is similar, the structures of their ion selectivity filters and their mechanisms of ion selectivity and conductance are completely different. Potassium channels select K+ by direct interaction with a series of four ion coordination sites formed by the backbone carbonyls of the amino acid residues that comprise the ion selectivity filter (Chapter 3). No charged amino acid residues are involved, and no water molecules intervene between K+ and its interacting backbone carbonyls in the ion selectivity filter of potassium channels. In contrast, the NavAb ion selectivity filter has a high field strength site at its extracellular end (see Figure 1-4, C; Glu177 = ε177), which is formed by amino acid residues that are highly conserved and are key determinants of ion selectivity in vertebrate sodium and calcium channels (see Figure 1-2). Considering its dimensions of approximately 4.6 Å2, Na+ with two planar waters of hydration could fit in this high–field-strength site. This outer site is followed by two ion coordination sites formed by backbone carbonyls (see Figure 1-4, D). These two carbonyl sites are perfectly designed to bind Na+ with four planar waters of hydration, but would be much too large to bind Na+ directly. In fact, the NavAb selectivity filter is large enough to fit the entire K+ channel ion selectivity filter inside it. Thus, the chemistry of Na+ selectivity and conductance is opposite to that of K+: negatively charged residues interact with Na+ to remove most (but not all) of its waters of hydration, and Na+ is conducted as a hydrated ion interacting with the pore through its inner shell of bound waters. Theoretical considerations of sodium selectivity and conductance predicted an outer high–field-strength site that would only partially dehydrate the permeating ion and two inner sites that would conduct and rehydrate the permeant Na+ ion because of the high energy of hydration of Na+ (see Figure 1-4, C, D).37 Figure 1-4 NavAb pore and selectivity filter. A, Architecture of the NavAb pore. Purple, Glu177 side-chains; gray, pore volume. B, The closed activation gate at the intracellular end of the pore illustrating the close interaction of Met221 residues in closing the pore. C, Top view of the ion selectivity filter. Symmetry-related molecules are colored white and yellow; P-helix residues are colored green. Hydrogen bonds between Thr175 and Trp179 are indicated by gray dashes. Electron densities from Fo-Fc omit maps are contoured at 4.0 σ (blue and gray), and subtle differences can be appreciated (small arrows). D, Side view of the selectivity filter. Glu177 (purple) interactions with Gln172, Ser178, and the backbone of Ser180 are shown in the far subunit. Fo-Fc omit map, 4.75 σ (blue); putative cations or water molecules (red spheres, IonEX). Electron-density around Leu176 (gray; Fo-Fc omit map at 1.75 σ) and a putative water molecule are shown (grey sphere). Na+-coordination sites: SiteHFS, SiteCEN and SiteIN. (Adapted from Payandeh J, Scheuer T, Zheng N, et al: The crystal structure of a voltage-gated sodium channel. Nature 475:353–358, 2011.) The voltage dependence of the activation of sodium channels derives from outward movement of approximately 12 gating charges as a consequence of depolarization of the membrane and reduction of the membrane electric field.25,38 The S4 segments of each homologous domain serve as the primary voltage sensors for activation.4,5 They contain repeated motifs of a positively charged amino acid residue followed by two hydrophobic residues creating a transmembrane spiral of positive charges. Upon depolarization, outward movement and rotation of S4 is thought to initiate a conformational change that opens the sodium channel pore.4,5 This “sliding helix” or “helical screw” model is supported by strong evidence. For example, neutralization of the positively charged residues in S4 reduce the voltage-dependence of gating.39 The outward and rotational gating movement of S4 segment has been detected directly by reaction of substituted cysteine residues in S4 segments with extracellular sulfhydryl reagents following channel activation and by analysis of the movement of fluorescent probes incorporated into these substituted cysteine residues.40,41 Other support for this mechanism derives from a wide range of structure and function studies.42 In the structure of the bacterial sodium channel NavAb, the S4 segment is in a transmembrane position in its activated state and its positive charges are thought to be neutralized by negative charges in the nearby S1 and S2 segments (Figure 1-5, A).21 At the resting membrane potential, the force of the electric field, which is negative inside the cell, would pull the positive charges inward. Depolarization would abolish this force and allow an outward movement of the S4 helix and its gating charges, catalyzed by exchange of ion pair partners (see Figure 1-5, B; Movie 1-1). After conformational changes have occurred in all four domains, the transmembrane pore can open and conduct ions (see Movie 1-1). This structural model shows that the S4 segment and its gating charges move through a narrow gating pore that focuses the transmembrane electric field to a distance of approximately 5 Å normal to the membrane and allows the gating charges to move from an intracellular aqueous vestibule to an extracellular aqueous vestibule with a short transit through the channel protein (see Video 1-1). Figure 1-5 The voltage-sensing domain (VSD). A, Side view of the VSD illustrating the extracellular negative charge-cluster (red; ENC), the intracellular negative charge-cluster (red; INC), hydrophobic constriction site (green; HCS), residues of the S1N helix (cyan), and phenylalanine of the S2-S3 loop (purple). S4 segment and gating charges (R1-R4) are in yellow. B, Transmembrane view of the lowest-energy Rosetta models of the VSD of NaChBac in Resting State 1 (left) and Activated State 3 (right). Side chains of the gating-charge-carrying arginines in S4 and key residues in S1, S2, and S3 segments are shown in stick representation and labeled. Gray, blue, and red atoms are C, N, and O, respectively. The HCS is highlighted by orange bars
Voltage-Gated Sodium Channels and Electrical Excitability of the Heart
Subunit Structure of Sodium Channels
Three-Dimensional Structure of Sodium Channels
Sodium Channel Structure and Function
Outer Pore and Selectivity Filter
Voltage-Dependent Activation
Stay updated, free articles. Join our Telegram channel
Full access? Get Clinical Tree
Voltage-Gated Sodium Channels and Electrical Excitability of the Heart
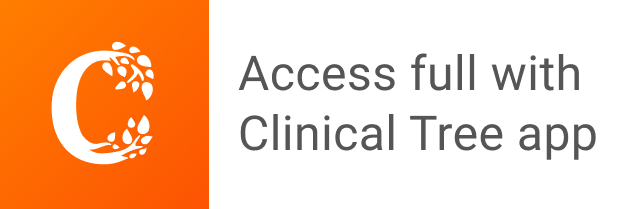