(1)
Project-team INRIA-UPMC-CNRS REO Laboratoire Jacques-Louis Lions, CNRS UMR 7598, Université Pierre et Marie Curie, Place Jussieu 4, 75252 Paris Cedex 05, France
Abstract
Blood and lymphatic vessels form networks for the transport of fluids, gases, macromolecules, particularly nutrients and signaling compounds, and circulating cells between the body’s tissues. The lymphatic network is involved in the immune surveillance of the body. Lymphocytes and antigen-presenting dendritic cells travel through lymphatics between peripheral tissues and lymphoid organs, where immunocytes mature.
Blood and lymphatic vessels form networks for the transport of fluids, gases, macromolecules, particularly nutrients and signaling compounds, and circulating cells between the body’s tissues. The lymphatic network is involved in the immune surveillance of the body. Lymphocytes and antigen-presenting dendritic cells travel through lymphatics between peripheral tissues and lymphoid organs, where immunocytes mature.
Blood and lymphatic networks are connected by left and right lymphatic ducts that drains lymph into blood circulation. Left lymphatic duct,1 or thethoracic duct, is the largest lymphatic vessel (length 38–45 cm; diameter ∼ 5 mm). It originates in the abdomen from the confluence of the right and left lumbar and intestinal trunks to terminate close to the junction of the left subclavian and left internal jugular veins. Lymph from the right arm, right region of the thoracic cavity, right side of the neck and head, and possibly lower left lobe of the left lung, is collected by the right lymphatic duct (length ∼ 12.5 mm), which commonly ends in the right subclavian vein, near its junction with the right internal jugular vein.
Both vascular structures are lined with endothelial cells that acquire tissue-specific specialization (Chap. 9). Endothelium ensures formation, maintenance, growth, and repair of vessel walls (Chap. 10).
7.1 Physical Environment
Vascular cells adapt to mechanical stimuli and remodel, thereby changing geometry, structure, and properties. Major stresses applied to blood vessels include: (1) axial stress induced by longitudinal tension; (2) circumferential stress (
: internal hydraulic radius; h:wall thickness [Laplacian equation for thin, homogeneous wall]) that results from perpendicularly applied blood pressure p i ; (3) radial compressive stress developed by blood pressure that has a much smaller magnitude with respect to axial and circumferential stresses; and (4) wall shear stress exerted by blood flow at the wetted surface of the vessel wall, i.e., friction on the luminal surface of the endothelium.
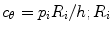
Stress distribution within large vessel wall is heterogeneous, as the wall is composed of 3 layers (intima, media, and adventitia) of composite materials. Moreover, stress field bears large temporal and spatial variations, as blood flow is three-dimensional, developing (or unestablished, as flow develops at the entrance of short curved vessels between 2 successive branchings),2 and unsteady.3
Elastic properties of vascular wall are usually represented by rheological parameters defined at specific pressures, as the pressure–cross-sectional area relationship is non-linear: (1) either vascular distensibility (
) or wall compliance and (2) elastic moduli (Vol. 7 – Chap. 5. Rheology). These parameters deal with the bulk wall behavior and do not represent elastic properties of wall material.

7.2 Vasculature Development
Mesodermal cells in the early embryo differentiate into endothelial precursors — angioblasts — and form blood islands. Fusion of blood islands leads to the primary capillary plexi. When blood circulation is established, primary plexi remodel into a hierarchical network of arteries, arterioles, capillaries, venules, and veins.
Vascular smooth myocytes (SMC) are associated with large and mid-sized vessels, whereas capillaries are covered by pericytes. First lymphatic endothelial cells sprout from embryonic veins, then they migrate to form lymphatic sacs. Lymphangiogenesis also involves sprouting, branching, and remodeling. Closed-end lymphatic capillaries drain into collecting vessels and ducts.
The branchial arch artery apparatus is a transient structure that appears in 6- to 7-week-old human embryos. Five branchial arches exist, each of which contains an arch artery (1–4 and 6). Blood exits from the heart via branchial arch arteries to circulate throughout the embryo. The branchial arch artery apparatus is initially symmetrical with equivalent amounts of blood flow through all of these arteries. This arterial apparatus afterward matures and sustains an asymmetrical remodeling. The left fourth and sixth arch arteries persist and generate the aortic arch and pulmonary trunk, whereas the right fourth and sixth branchial arch arteries regress, as blood predominantly flows through the left arch arteries.
This event is concomitant with increased expression of growth factors, such as platelet-derived (PDGF) and vascular endothelial (VEGF) growth factors. It follows rotation and realignment of the ventricular outflow tract that forms part of the left and right ventricles. Outflow tract rotation is required for reshaping of the right sixth arch artery into a long, narrow vessel with reduced blood flow.
The transcription factor pituitary paired-like homeodomain-containing protein Pitx2 (encoded by the homeobox PITX2 gene) stimulated by Nodal, a member of the TGFβ growth factor superfamily involved in cell differentiation, mesoderm formation, and subsequent organization of left–right axial structures, regulates the asymmetric growth. It abounds in cells of the secondary heart field that includes the myocardium of the left wall of the outflow tract. Pitx2 + cells participate to the control of rotation of the outflow tract. Factor Pitx2 is not synthesized in cell types that belongs to or surrounds sixth branchial arch arteries [652]. Although branchial arch artery remodeling requires Pitx2, it is not directly governed by Pitx2 + cells. The anterior heart also requires FGF8 that is overexpressed in the absence of Pitx2 protein. Factor FGF8 may be involved in arch artery maturation. Besides, the Nodal–Pitx2 pathway and abnormal rotation of the outflow tract do not strongly affect branchial arch artery laterality.
Vascular growth factors, such as PDGFa protomer and VEGF, contribute to branchial arch artery apparatus remodeling, as their inhibition specifically yields loss of both left and right sixth arch arteries [652]. Expression of PDGFa is maintained on the left side but decays on the right. The concentration of phosphorylated VEGFR2 in endothelial cells also decreases in the right sixth branchial arch artery, whereas in the case of Pitx2 loss, PDGFa and VEGFR2P levels remain symmetrical. In addition, ligated left sixth branchial arch artery in 11.5-day-old mouse embryos (before the onset of remodeling; i.e., approximately 35-day-old human embryos) leads to their regression and development of right arteries [652]. In 4 among 6 embryos with ligated left sixth branchial arch artery, PDGFR and VEGFR2P signalings are reduced on the left side and maintained on the right side. Therefore, hemodynamical stress shapes the asymmetrical arterial system by operating via growth factors.
7.3 Wall Structure
As blood pulses in an artery, its wall alternatively stretches and recoils. In 1733, S. Hales described blood systolic storage and diastolic restitution. In 1899, O. Frank modeled arterial capacitance by awindkessel element, i.e., a damping air chamber incorporated in a rigid pipe network. Blood vessels are pressure and volume buffers. Veins that are the major blood storage compartments, accommodate volume changes by shrinking to possibly reach a collapse state.
Wall expansion and relaxation depend on the rheology of the vessel wall, and thus on its composition and structure. In particular, distension and subsequent recoil are achieved by the architecture and interactions between coupledelastin andcollagen fibers. In addition to passive caliber changes, the vessel wall undergoes active variations due tovasomotor tone. Vascular smooth muscle activity fits the blood flow to the tissue requirements.
Structural components exist in every kind of blood vessel, except capillaries, although the element amount and structure vary between vessel types.Endothelial cells with a hemodynamic stress-dependent shape (50–100 ×10 ×0.5–2 μm), line the blood–wall interface of the whole vasculature (Chap. 9 ).Smooth myocytes are responsible for vessel tone and hence lumen size (Chap. 8). They thus influence the vascular impedance.
Specific cells of connective tissue (Vol. 1 – Chap. 1. Cells and Tissues) includefibroblasts (FB; Vol. 1 – Chap. 2. Cells of the Blood Circulation)) that produce ground matrix and fibers in growing tissues andfibrocytes (FC). The ground matrix fills the space between cells and fibers. It is composed of interstitial fluid that contains electrolytes and plasma molecules,proteoglycans, and glycoproteins. Elastin forms low-stiffness elastic fibers, taut in the unstretched configuration that can stretch up to 60% with elastic behavior. Collagen makes high-stiffness fibers that are tortuous in the unstretched configuration and are responsible for structural integrity. Thereby, elastin provides vessel distensibility and collagen tensile strength.4
The wall structure is characterized by a tunica set that comprises intima, media, and external adventitia in large arteries and veins (Fig. 7.1). Tunica number and structure vary according to vessel type and size.
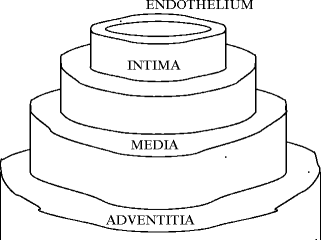
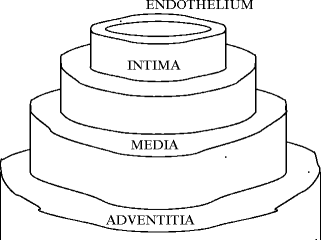
Fig. 7.1
Wall tunicae. The media (intermediate layer) is bounded by the elasticae interna and externa from the intima (inner layer) and adventitia (outer layer).
7.3.1 Large Vessels
The wall of large blood vessels has a circumferentially layered structure. The internal thin intima is composed of the inner endothelium and a subendothelial tunica of connective tissue. The endothelium is a monolayer of endothelial cells supported at its abluminal surface by a basal lamina. The endothelium modulates wall structure and functions.
The internal elastic lamina (IEL) made of numerous azimuthal bundles of elastic fibers delimits the intima from the media. The fenestrated internal elastic lamina is characterized by a quasi-uniform distribution of pores. Water and solutes are transported through these fenestrae. Cells communicate and migrate via these pores. The number of fenestrations is greater at branching points.
The middle muscular tunica — media — is formed by layers of circumferentialsmooth myocytes and connective tissue with azimuthally arranged elastic fibers. Elastin sheets, or lamellae, are fenestrated to facilitate material diffusion. Action potentials trigger SMC contraction after a delay ranging from 80 to 100 ms that lasts from 10 to 15 s [653]. According to the vessel type, media contains varying amounts of collagen and elastic fibers, elastic lamellae, and proteoglycans secreted by smooth myocytes. Media of arteries is larger than that of veins of similar size.
The external elastic lamina (EEL), a thick elastic circumferential band, is located between the media and adventitia.
The external adventitia consists mainly of connective tissue with predominant fibroblasts, some smooth myocytes, many macrophages, elastic fibers and predominant longitudinally arranged collagen fibers. In large vessels, the adventitia contains nerves, vessels — vasa vasorum, and possibly lymphatic vessels. Media of arteries is much thinner than that of veins of similar size.
7.3.1.1 Elastic Arteries
The media is the main site of histological specializations of artery walls (Table 7.1). Vessels proximal to the heart are elastic arteries.
Table 7.1
Artery wall structure. Downstream from the heart, the arterial tree successively comprises elastic, transition, and muscular arteries, and arterioles. The different artery types act as conducting, distributing, and resistive arteries with increasing distance from the heart. During the systole, elastic arteries store a part of the blood volume ejected by the left ventricle for diastolic restitution owing to wall recoil. Peripheral muscular arteries and arterioles locally control the lumen size and the vessel resistance.
Layer | Elastic | Muscular | Arteriole | Cerebral |
---|---|---|---|---|
artery | artery | artery | artery | |
Caliber | Great | Mid | Small | Mid |
Intima | Absence of | |||
subendo- | ||||
thelium | ||||
IEL | Thin | Important, | Thin | Well |
elastic | scalloped | Thin | developed | |
lamellae | ||||
Media | Thick | Thin | 1–5 layers | Almost |
Numerous | Numerous | of SMCs | no EnFs | |
elastic | layers | |||
lamellae | of SMCs | |||
EEL | Badly | Clearly | Badly | Absent |
defined | defined | defined | ||
Adventitia | Thin | Thick |
Wall distension during pressure wave propagation for efficient windkessel effect is facilitated by thin concentric fenestrated lamellae5 of elastin in a thick media, between which are smooth myocytes and collagen fibers (Table 7.2). The lamella number depends on vessel size, between-lamella space being nearly constant. Inside the elastic lamella, elastic fibers have a helical orientation.6
Table 7.2
Wall structure (EC: endothelial cell; FB: fibroblast; SMC: smooth muscle cell; Col: collagen; Eln: elastin; FN: fibronectin; Lam: laminin; PoG: proteoglycan; Tsp: thrombospondin; vWF: von Willebrand factor).
Wall layer | Components |
---|---|
Endothelium | EC |
Basement membrane | Col4, Lam |
Subendothelium | Col3/4/5, Lam, FN, PoG, Tsp, vWF, |
FB, ± SMC | |
IEL | Eln |
Media | Col, Eln, PoG, |
FB, SMC | |
EEL | Eln |
Adventitia | FB, Eln, Col |
Vasa vasorum, nerve endings, lymphatics |
The external elastic lamina is not very well defined and the adventitia is thinner. The orientation and extension of the collagen fibers of the adventitia of human aortas that undergo uniaxial tension are correlated to the applied stress [654]. After straightening and suitable orientation, collagen fibers can bear increasing loads.
7.3.1.2 Muscular Arteries
Muscular arteries have thinner intima. The media is characterized by numerous concentric layers ofsmooth myocytes with elastic fibers and few collagen fibers. The external elastic lamina can be clearly observed. The wall content in elastin and collagen varies according to the artery type, elastic, transitional, or muscular (Table 7.3). The fibers have a helical orientation with a variable pitch angle from the inner to the outer vessel wall. Cerebral arteries are thin walled without external elastic lamina.
Collagen | Elastin | |
---|---|---|
Ascending aorta | 15–20 | 40–50 |
Thoracic aorta | 15–30 | 20–40 |
Abdominal aorta | 25–35 | 15–20 |
Femoral artery | 35–45 | 15–25 |
Elastic modulus | 102–106 | 102–103 |
Adventitia that surrounds most blood vessels allows cell migration into and out of the artery wall. Adventitial cells participate in vascular growth and remodeling. Adventitia is a residence zone for progenitor cells that can adopt both vascular and non-vascular fates. Endothelial progenitor cells form clusters between the media and adventitia in arteries.
The morphogenSonic hedgehog (Vol. 3 – Chap. 10. Morphogen Receptors) stimulates production of angiogenic factors (e.g., VEGFa and angiopoietin-1) by fibroblasts, and promotes angiogenesis. It also specifies arterial or venous identity of endothelial cells and recruits mural cells. Sonic Hedgehog restricted to the adventitia of artery wall favors maintenance of resident SCA1 + vascular progenitor cells [657].7 Some progenitors self-renew, whereas others differentiate.
These progenitors express transcription factors for SMC differentiation, includingserum response factor andmyocardin family members8 (i.e., myocardin and myocardin-related transcription factors MRTFa and MRTFb), as well as transcriptional corepressors of the SRF-dependent transcription (Krüppel-like factor KLF4,homeodomain-containing protein Hox7,9 andforkhead box transcription factor FoxO4) for progenitor maintenance.
7.3.1.3 Veins
Vein walls are thinner than artery walls, whereas the bore is larger (Table 7.4). The intima is very thin. Internal and external elastic laminae are either absent or very thin. The media is thinner than the adventitia.
Table 7.4
Comparison between a vein and its associated artery walls.
Artery | Vein | |
---|---|---|
Shape | Circular | Elliptical |
Wall | Thick | Thin |
Intima | Very thin | |
Media | Prominent | Weak |
IEL | Quasi-absent |
Medium-sized veins are characterized by the presence of valves to prevent transient blood return to upstream segments during muscular compression (Figs. 7.2 and 7.3).
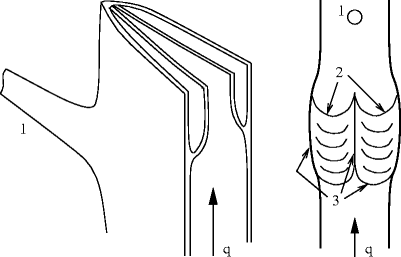
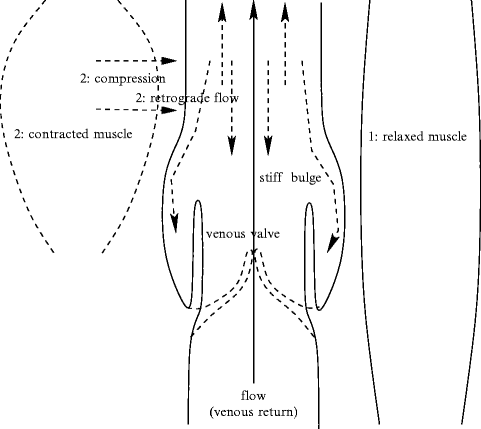
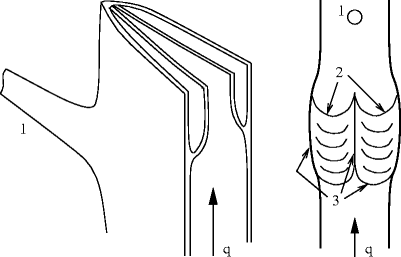
Fig. 7.2
Bicuspid venous valves. (Left) Cross and axial cut of a vein. (Right) Open and spread vein after axial incision of a wall edge (1: orifice of communication vein at the vein edge; 2: cusp free border in front of the vein face; 3: valve insertion lines). The valvular sinuses are behind the cusps. The luminal face is smooth; the parietal face is rough.
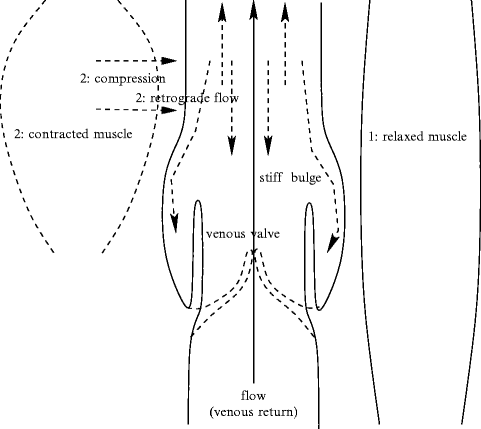
Fig. 7.3
Venous valves are fully open when neighboring muscles are relaxed. Adjoining muscle contraction compresses many more avalvular segments of veins than valvar parts that are slighly dilated and stiffer. Consequently, venous return is favored, but is associated with retrograde flow. The latter is impeded by valve closure due to blood stream between the vein wall and the valve.
The largest veins of the abdomen and thorax have very thick adventitia that contains bundles of longitudinal SMCs and vasa vasorum. Valves are absent. The main features of the wall are given for different kinds of veins, sorted by their bore, in Table 7.5.
Table 7.5
Vein wall structure.
Vein type | Caliber | Wall |
---|---|---|
Microvenule | 15–100 μm | Endothelium, |
thin layer with CnFs, ± FBs, | ||
SMCs if caliber > 50 μm | ||
Minivenule | 100–300 μm | Continuous layer of SMCs, |
± postcapillary sphincter | ||
Venule | 300–500 μm | Media, |
thin adventitia (EnFs, CnFs, FBs) | ||
Drainage vein | 0.5–2 mm | Intima with subendothelium, |
± EnF network, | ||
adventitia | ||
Collecting vein | 2–9 mm | Intima sometimes bounded |
by EnF network, | ||
media poorly developed, | ||
except in inferior limbs, | ||
thick adventita, | ||
valves in zones of | ||
greater caliber | ||
Central vein | 20–30 mm | Adventitia of vena cava |
with CMCs in the heart vicinity |
Different gene expression occurs between arterial and venous walls that are exposed to different mechanical stress magnitudes. γ-Catenin (or plakoglobin), galactoside-binding, soluble lectin LGalS7 (orgalectin-7),10 sciellin (Scel), and small proline-rich protein SPrR3,11 are expressed in arteries but not veins [658]. γ-Catenin, sciellin, SPrR3, and galectin-7, as well as periplakin (PPl)12 and envoplakin (EvPl),13 are arterial intima-enriched components [659].14
7.3.1.4 Venous Valves
Perforating veins usually contain venous valves that prevent reflux of blood from the deep veins into the superficial veins(ostial valve).
Although venous drainage in the human head and neck commonly does not counter gravity, venous valves are also found throughout veins of these body parts [660].
Vieussens valve is the ostial valve of the great cardiac vein located near the coronary sinus that is provided at its ostium by theThebesian valve [661].
Venous valves most often are bicuspid and located in stiffer expanded segments (Figs. 7.2 and 7.4). Venous valves are made of connective tissue with elastic fibers and few smooth myocytes, covered by the endothelium.
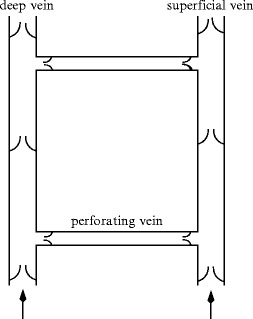
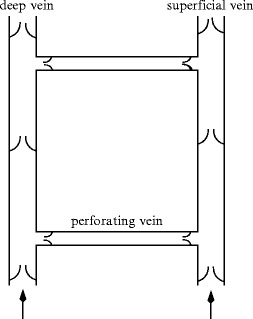
Fig. 7.4
Limb valved vein network, with a superficial vein and a deep vein anastomosed by a communicating vein equipped with ostial valves for one-way circulation from superficial to deep vein.
Veins usually have elliptical cross-sections. Valve leaflets are inserted on each face of the vein wall,15 whereas vein edges receive perforating and tributary veins [665].
Endothelial cell density is significantly higher on the surface of valve leaflets (about 10 cells per 1000 μm2) than on other venous surfaces (about 7 cells/1000 μm2) [666]. Endothelial cells on the medial surface and on the lateral surface of the valve leaflet are oriented parallel to the vein axis and perpendicular to it, respectively.
7.3.2 Small Vessels
Small blood vessels of the microcirculation comprise perfusing arterioles, capillaries (caliber 5–10 μm), and draining venules (caliber 8–100 μm).
7.3.2.1 Arterioles
Arterioles are composed of an endothelium surrounded by one or a few concentric layers of smooth myocytes that regulate blood flow (Table 7.6). Arterioles thus have a thin wall. Arterioles receive both sympathetic and parasympathetic innervation.
Table 7.6
Wall structure difference in blood macro- and microcirculation (ColF: collagen fiber; EC: endothelial cell; ElnF: elastin fiber; FB: fibroblast; vSMC: vascular smooth muscle cell).
Macrocirculation | Microcirculation |
---|---|
Intima | Endothelium |
Endothelium (glycocalyx) | (monolayer of ECs, |
Subendothelium (vSMC, ElnF) | blood–wall interface) |
IEL | |
Media (vSMC, ElnF) | 1–2 layers of vSMC in arterioles, |
pericytes in capillaries | |
EEL | Elastic externa ( ± ) |
Adventitia (FB, ElnF, ColF) | Absent in arterioles |
Nervi vascularis (end on vSMC) | |
Vasa vasorum (cap plexi) |
7.3.2.2 Capillaries
Capillaries16 are small exchange vessels composed of endothelium surrounded by a basement membrane.
A capillary bed can encompass 2 types of vessels: (1) true capillaries which branch mainly from metarterioles17 and yield mass exchange between cells and blood and (2) a short vascular shunt that directly connects the arteriole and venule at opposite ends of the capillary bed.
Three structural types exist. Continuous capillaries in muscles, skin, lung, and central nervous system are defined by a continuous basement membrane and tight intercellular clefts and have the lowest permeability. Fenestrated capillaries in exocrine glands, renal glomeruli, and intestinal mucosa are characterized by perforations in endothelium and thus by relatively high permeability. Discontinuous capillaries in the liver, spleen, and bone marrow, are defined by large intercellular and basement membrane gaps and, consequently, very high permeability.
Precapillary sphincters are rings of smooth myocytes at the origin of true capillaries that regulate blood flow into tissues. Mass flux across capillaries depends mainly on 6 variables: (1) capillary and interstitial hydrostatic pressure; (2) capillary and interstitial oncotic pressure; and (3) filtration and reflection coefficients.
7.3.2.3 Venules
Many venules unite to form a vein. Venules are composed of an endothelium surrounded by a basement membrane for the postcapillary venules and smooth muscle for the larger venules (Table 7.5). Larger venules possess a middle layer of muscular and elastic tissue and an outer tunica of fibrous connective tissue. Venules have thinner walls than arterioles.
High endothelial venules (HEV) are specialized postcapillary venules characterized by simple cuboidal cells. They enable circulating lymphocytes to enter a lymph node.
7.4 Blood–Brain Barrier
Vascular endothelial cells in the central nervous system form a barrier that restricts molecule fluxes between the blood and brain. Thisblood–brain barrier (BBB) ensures proper neuronal functioning and protects the central nervous system (CNS). The blood–brain barrier results from interactions between endothelial cells and neural cells. Its main constituents include endothelial cells, astrocytes, and pericytes, in addition to the extracellular matrix.
The average distance fromcapillary to neuron ranges from 8 to 20 μm. The microvasculature of the central nervous system is aimed, in addition to its usual goals, at maintaining the homeostasis of the internal medium. A constant microenvironment is indeed required for neuronal activity.
The blood–brain barrier was first described by P. Ehrlich in 1903. It corresponds to a specialized endothelium of cerebral capillaries that avoids any fluctuation in substance concentration in the extracellular space, especially ions, whereas blood supplies the brain with the required nutrients (Fig. 7.5). Unlike peripheral capillaries that allow substance filtration across and between endothelial cells, the blood–brain barrier strictly limits transport into the brain through cerebral microvascular endothelial cells sealed by tight junctions18 (Vol. 1 – Chap. 7. Plasma Membrane) and plasmalemmal carriers. The endothelial cells of the blood–brain barrier are characterized by transport restriction due to tight junctions and polarized carriers, as well as unique metabolic properties.
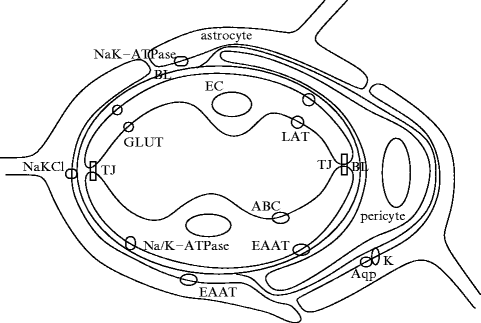
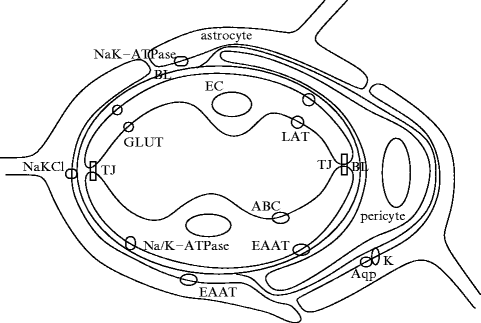
Fig. 7.5
Schematic drawing of the blood–brain barrier, with endothelial cells (EC) of the cerebral capillary, surrounded by basal lamina (BL), perivascular extensions of astrocytes and pericytes (Source: [667]). Endothelial cells are joined by tight junctions (TJ). Endothelium contains carriers for glucose (glucose transporter [GLUT]), amino acids (L system for large neutral amino acids [LAT]), glutamate (excitatory amino acid transporters [EAAT]), ions (Na/K-ATPase), and ABC transporters. Astrocyte extensions facing the capillary wall have patches with aquaporins (Aqp4) and K + channels (K). They contain also Na + –K + ATPase, Na + –K + –Cl − cotransporters, and EAATs.
Walls of cerebral capillary is thinner than that of the muscle capillary [668]. The wall thickness could modulate the restrictive permeability of the blood–brain barrier with a shorter transendothelium transport time. A greater mitochondrion number might exist in BBB endothelial cells than in other endothelial cells, in relation to energy-dependent transcapillary transport [669].
The restrictive blood–brain barrier with its enzymes and transporters develops during embryogenesis. Endothelial cells characterized by a reduced vesicular transport form nascent vessels in the central nervous system to which pericytes are recruited, before astrocytes are generated [678].Pericytes regulate the function and proper structure of the blood–brain barrier, in particular the formation of tight junctions and vesicle transfer in endothelial cells of the central nervous system [678]. They inhibit the expression of molecules that increase vascular permeability and immunocyte infiltration. Pericytes reduces the permeability of the blood–brain barrier to water and low- and high-molecular-mass molecules [671]. In addition, pericytes may mediate the attachment of astrocyte end-feet to endothelial cells [671].The PDGFb–PDGFRβ complex is required in pericyte recruitment during angiogenesis.
7.4.1 BBB Restricted Permeability
The brain endothelium has a much lower capacity for endo- and transcytosis than that of peripheral endothelia. Furthermore, intra- and extracellular enzymes yield a metabolic barrier. Ectoenzymes, such as peptidases and nucleotidases, are able to metabolize peptides and ATP, respectively. Intracellular enzymes such as monoamine oxidase and cytochrome-P450 can inactivate many neuroactive and toxic compounds. Renin, angiotensin-converting enzyme, and aminopeptidase-A acting on angiotensin belong to degrading enzymes of the blood–brain barrier. Certain substances that are not carried by the endothelium, are metabolized in endothelial cells such that they cannot enter into the brain. The cerebral endothelium has enzymes that mainly target neuroactive substances, found in low concentration or absent in other capillaries.
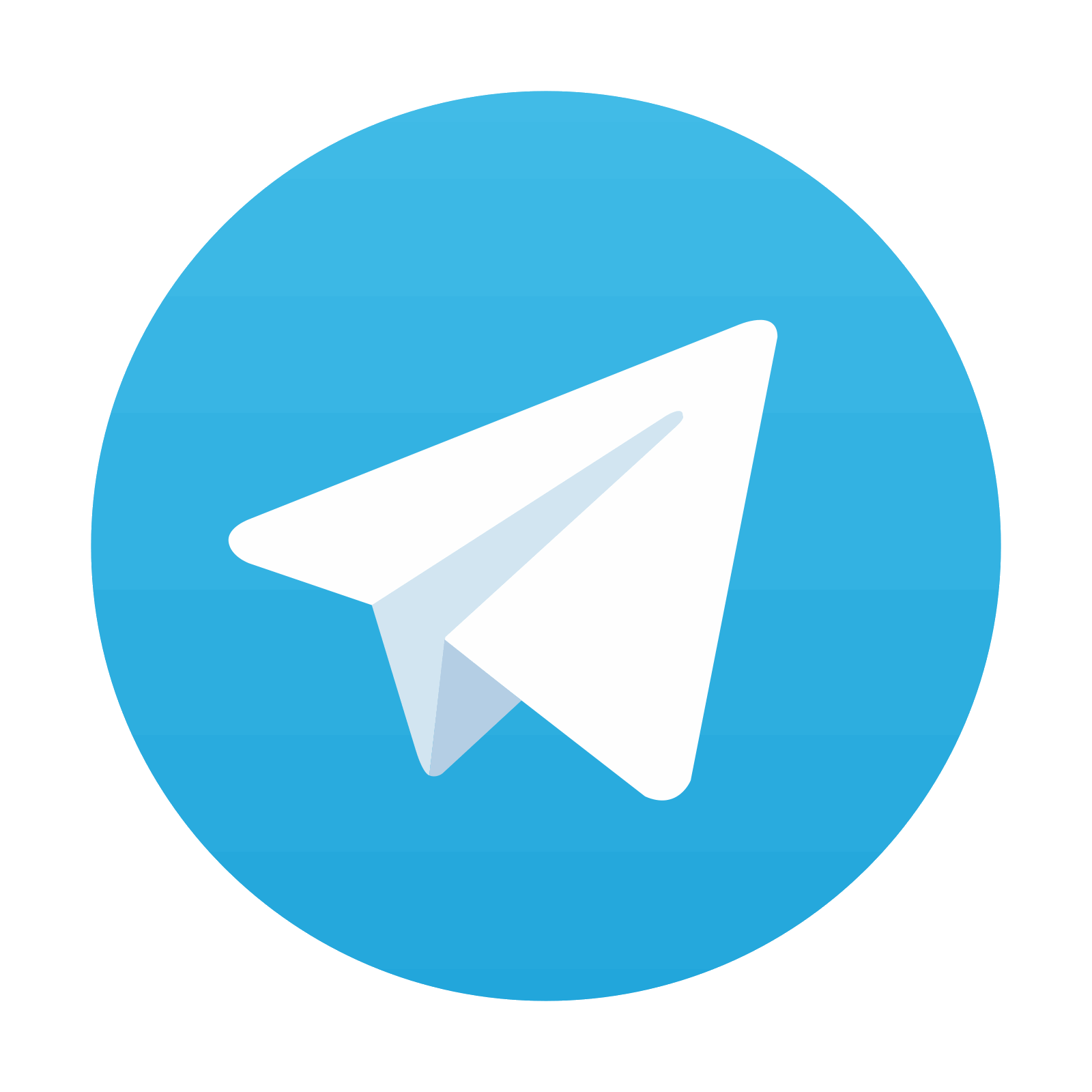
Stay updated, free articles. Join our Telegram channel
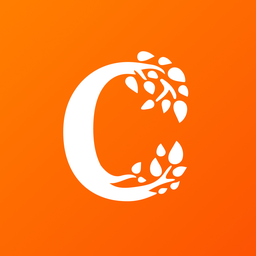
Full access? Get Clinical Tree
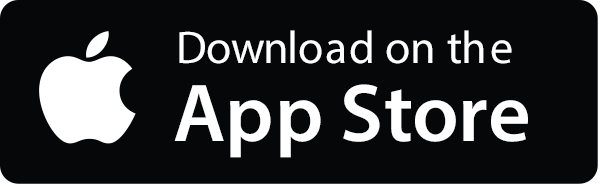
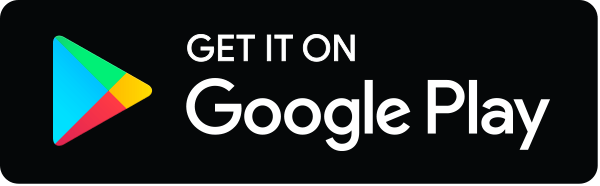