Abstract
The majority of sudden cardiac death (SCD) events are associated with structural heart disease; however, in 10% to 20% of SCDs, no cardiac structural abnormalities are detectable. The lack of an apparent cause in many of those cases initially led to the classification as “sudden unexplained death syndrome” or “sudden infant death syndrome.” Many of these are caused by primary electrical disorders, including long QT syndrome, catecholaminergic polymorphic VT, Brugada syndrome, and short QT syndrome, as well as cases identified as “idiopathic VF” when the underlying cause often remains unknown.
Risk stratification, genetic testing, genetic counseling, lifestyle modifications, pharmacologic therapy, and defibrillator implantation are important considerations in the management of affected individuals.
Keywords
inherited channelopathies, long QT syndrome, Brugada syndrome, catecholaminergic polymorphic ventricular tachycardia, short QT syndrome, idiopathic VF, early repolarization syndromes
Outline
Long QT Syndrome, 976
Genetics of the Long QT Syndrome, 977
Pathophysiology of the Long QT Syndrome, 980
Epidemiology, 983
Clinical Presentation and Natural Course, 983
Electrocardiographic Features, 985
Diagnosis of the Long QT Syndrome, 989
Differential Diagnosis: Acquired Long QT Syndrome, 992
Risk Stratification, 994
Principles of Management, 996
Brugada Syndrome, 1000
Genetics of the Brugada Syndrome, 1000
Pathophysiology of the Brugada Syndrome, 1002
Epidemiology, 1004
Clinical Presentation, 1004
Electrocardiographic Features, 1005
Diagnosis of the Brugada Syndrome, 1008
Differential Diagnosis, 1009
Risk Stratification, 1011
Principles of Management, 1013
Short QT Syndrome, 1017
Genetics of the Short QT Syndrome, 1017
Pathophysiology of Short QT Syndrome, 1018
Epidemiology, 1018
Clinical Presentation, 1018
Electrocardiographic Features, 1018
Diagnosis of the Short QT Syndrome, 1019
Differential Diagnosis, 1019
Risk Stratification, 1019
Principles of Management, 1019
Catecholaminergic Polymorphic Ventricular Tachycardia, 1020
Genetics of Catecholaminergic Polymorphic Ventricular Tachycardia, 1020
Pathophysiology of Catecholaminergic Polymorphic Ventricular Tachycardia, 1020
Epidemiology, 1022
Clinical Presentation, 1022
Electrocardiographic Features, 1022
Diagnosis of Catecholaminergic Polymorphic Ventricular Tachycardia, 1022
Differential Diagnosis, 1024
Risk Stratification, 1024
Principles of Management, 1024
Idiopathic Ventricular Fibrillation, 1025
Genetics of Idiopathic Ventricular Fibrillation, 1025
Pathophysiology of Idiopathic Ventricular Fibrillation, 1026
Epidemiology, 1026
Clinical Presentation, 1026
Electrocardiographic Features, 1026
Diagnosis of Idiopathic Ventricular Fibrillation, 1026
Principles of Management, 1028
Early Repolarization Syndromes, 1029
Genetics of Early Repolarization Syndrome, 1029
Pathophysiology of Early Repolarization Syndromes, 1030
Epidemiology, 1031
Clinical Presentation, 1031
Electrocardiographic Features, 1031
Diagnosis of Early Repolarization Syndrome, 1034
Differential Diagnosis, 1034
Risk Stratification, 1034
Principles of Management, 1037
Sudden cardiac death (SCD) is a major contributor to population mortality, with an overall incidence in the United States estimated to be between 0.1% and 0.2%, resulting in approximately 300,000 to 350,000 deaths annually. Ventricular fibrillation (VF) or pulseless ventricular tachycardia (VT) is the initial rhythm recorded in 25% to 36% of witnessed cardiac arrests occurring at home, but in a much higher proportion (38% to 79%) of witnessed cardiac arrests occurring in a public setting. The majority of SCD events are associated with structural heart disease, with coronary artery disease and its complications being involved in up to 60% to 80% of cases, followed by other cardiomyopathies. However, in 10% to 20% of SCDs, no cardiac structural abnormalities are detectable. The lack of an apparent cause in many of those cases initially led to the classification as “sudden unexplained death syndrome” (SUDS) or “sudden infant death syndrome” (SIDS). Many of these are caused by primary electrical disorders, including long QT syndrome (LQTS), catecholaminergic polymorphic VT (CPVT), Brugada syndrome, and short QT syndrome (SQTS), as well as cases identified as “idiopathic VF” when the underlying cause often remains unknown.
Long QT Syndrome
The LQTS is an uncommon inherited cardiac channelopathy that is associated with an abnormally prolonged QT interval and an increased propensity for life-threatening ventricular arrhythmias in the presence of a structurally normal heart.
In 1957, Anton Jervell and Fred Lange-Nielsen published the first report on a familial (autosomal recessive) disorder (“Jervell and Lange-Nielsen syndrome”) characterized by the presence of a striking prolongation of the QT interval, congenital deafness, and a high incidence of SCD at a young age. Subsequently, Romano and Ward independently identified an almost identical, but autosomal dominant, disorder that is not associated with deafness (“Romano-Ward syndrome”). A genetic relationship between the two was then proposed and the two syndromes were considered variants of one disease under the unifying name of “LQTS.” In the contemporary literature, Romano-Ward syndrome is used interchangeably with LQTS, but is now less commonly used in favor of the LQT1 to LQT17 scheme according to the underlying genetic mutation (see later).
The initial molecular studies suggested that all genes linked to the LQTS phenotype encode for various subunits of cardiac ion channels. Subsequent findings, however, revealed that LQTS could also be caused by mutations of gene coding for channel-associated cellular structural proteins as well. Nonetheless, the concept that LQTS genes ultimately affect cardiac ion currents, either directly (ion channel mutations) or indirectly (modulators), still holds true.
Genetics of the Long QT Syndrome
To date, more than 600 mutations of 17 different genes responsible for a hereditary form of LQTS have been identified (LQT1-17) ( Table 31.1 ; Fig. 31.1 ), with the majority (more than 90%) of the known mutations located in the first three genes: LQT1 (KCNQ1) mutations account for 40% to 55% of genetically positive LQTS, LQT2 (KCNH2) for 30% to 45%, and LQT3 (SCN5A) for 5% to 10%.
Disease | Gene | Protein | Functional Effect | Frequency |
---|---|---|---|---|
LQT1 | KCNQ1 (KvLQT1) | K v 7.1 | ↓I Ks | 40%–55% |
LQT2 | KCNH2 (HERG) | K v 11.1 | ↓I Kr | 30%–45% |
LQT3 | SCN5A | Na v 1.5 | ↑I Na | 5%–10% |
LQT4 (Ankyrin-B syndrome) | ANKB | Ankyrin-B | Aberrant ion channel/transporter localization | <1% |
LQT5 | KCNE1 | MinK | ↓I Ks | <1% |
LQT6 | KCNE2 | MiRP1 | ↓I Kr | <1% |
LQT7 (Andersen-Tawil syndrome) | KCNJ2 | Kir2.1 | ↓I K1 | <1% |
LQT8 (Timothy syndrome) | CACNA1C | Ca v 1.2 | ↑I CaL | <1% |
LQT9 | CAV3 | Caveolin 3 | ↑ I Na | <1% |
LQT10 | SCN4B | Na v β4 | ↑I Na | <1% |
LQT11 | AKAP9 | Yotiao | ↓I Ks | <1% |
LQT12 | SNTA1 | Syntrophin-α1 | ↑I Na | <1% |
LQT13 | KCNJ5 | Kir3.4 (GIRK4) | ↓I KACh | <1% |
LQT14 | CALM1 | Calmodulin 1 | ↑I CaL | 1%–2% |
LQT15 | CALM2 | Calmodulin 2 | ↑I CaL | <1% |
LQT16 | CALM3 | Calmodulin 3 | ↑I CaL | <1% |
LQT17 (Triadin knockout syndrome) | TRDN | Triadin | ↑I CaL | 2% |
JLN1 | KCNQ1 (KvLQT1) | K v 7.1 | ↓I Ks | Very rare |
JLN2 | KCNE1 | MinK | ↓I Ks | Very rare |

Overall, nine of these genes encode ion channel subunits that are specifically involved in cardiac action potential generation. LQT4, LQT9, LQT11, LQT12, and LQT14-LQT17 are caused by mutations in a family of versatile membrane adapters other than ion channel subunits.
The majority of LQTS cases are inherited in an autosomal dominant fashion. Conversely, Jervell and Lange-Nielsen syndrome, which is inherited in an autosomal recessive fashion, is very rare, affecting less than 1% of LQTS cases.
Genetic analysis reveals two or more mutations in 8% to 11% of LQTS patients with clinical phenotypes of autosomal dominant Romano-Ward syndrome. These compound mutations (so-called “double hits”) appear to be associated with a more severe phenotype than that associated with a single hit.
Most reported LQTS genetic mutations occur in coding regions, although noncoding mutations (resulting in the loss of allele expression) have also been described. Most LQTS families have their own mutations, which are often termed “private” mutations.
Several genetic mechanisms have been implicated in the development of LQTS including abnormalities in protein synthesis (transcription, translation), posttranslational protein processing resulting in abnormal transport to the cell surface membrane (protein trafficking, folding, assembly of subunits, glycosylation), ion channel gating (biophysical and kinetic properties), or permeation (ion selectivity, unitary conductance).
The majority of LQTS cases are caused by heterozygous disease; thus mutations causing abnormalities in channel coassembly or trafficking result in up to 50% maximal reduction in the number of functional channels (haplotype insufficiency), because the gene product from the healthy allele remains intact. On the other hand, mutations that abolish channel function while preserving subunit assembly can result in dominant-negative suppression of the healthy allele as well, causing a more severe reduction (up to 94%) of the total amount of functional protein (dominant-negative effect) and favoring a more severe clinical course and a higher frequency of arrhythmia-related cardiac events.
Mutations Related to the Potassium Current
Mutations related to the slowly activating delayed rectifier potassium current.
Slowly activating delayed rectifier potassium current (I Ks ) contributes to human atrial and ventricular repolarization, particularly during action potentials of long duration, and plays an important role in determining the rate-dependent shortening of the cardiac action potential. Mutations in LQT1, LQT5, and LQT11 result in attenuation of I Ks and, as a consequence, prolongation of repolarization, action potential duration and QT interval. LQT1 is caused by loss-of-function mutations of the KCNQ1 (KvLQT1) gene, which encodes the α subunit (K v 7.1) of the inward I Ks . More than 170 mutations of this gene have been reported, comprising many Romano-Ward (autosomal dominant) syndromes and accounting for approximately 40% to 55% of all genotyped LQT families. Of note, mutations involving the transmembrane domain of KCNQ1 result in more severe disease compared with C-terminal mutations. LQT5 is caused by loss-of-function mutations of the KCNE1 gene, which encodes the β- subunit (MinK) that modulates I Ks .
Homozygous or compound heterozygous loss-of-function mutations of either the KCNQ1 or KCNE1 genes cause the autosomal recessive form of LQTS (the Jervell and Lange-Nielsen syndrome). Patients with KCNQ1 mutations (type 1 Jervell and Lange-Nielsen syndrome) have an almost sixfold greater risk of arrhythmic events, whereas patients with KCNE1 mutations (type 2 Jervell and Lange-Nielsen syndrome) appear to be at lower risk. Although the Jervell and Lange-Nielsen syndrome is the most severe among the major variants of LQTS, the parents of Jervell and Lange-Nielsen syndrome patients are generally less symptomatic than other LQT1 patients, despite the fact that they all are heterozygous for the same gene. This is likely related to the observation that most of the LQT1 genetic variants are missense mutations exerting a dominant-negative effect, whereas most (74%) Jervell and Lange-Nielsen mutations of KCNQ1 are frame-shift/truncating mutations that are unable to cause dominant-negative suppression but are likely to interfere with subunit assembly. Jervell and Lange-Nielsen syndrome accompanies complete loss of I Ks in hair cells and endolymph of the inner ear, which results in congenital deafness.
LQT11 is caused by loss-of-function mutations of the AKAP9 gene, which encodes an A-kinase anchoring protein (Yotiao), shown to be an integral part of the I Ks macromolecular complex. The presence of Yotiao is necessary for the physiological response of the I Ks to beta-adrenergic stimulation. LQT11 mutations reduce the interaction between Yotiao and the I Ks channel (K v 7.1), preventing the functional response of I Ks to cyclic adenosine monophosphate (cAMP) and adrenergic stimulation and causing an attenuation of I Ks .
Mutations related to the rapidly activating delayed rectifier potassium current.
Rapidly activating delayed rectifier potassium current (I Kr ) presents the principal repolarizing current at the end of the plateau phase in most cardiac cells and plays an important role in governing the cardiac action potential duration and refractoriness. Mutations in LQT2 and LQT6 result in attenuation of I Kr and cause a decrease in the K + outward current and prolongation of repolarization, action potential duration, and QT interval.
LQT2 is caused by loss-of-function mutations of the KCNH2 (HERG) gene, which encodes the α-subunit (K v 11.1) of the inward I Kr . LQT2 syndrome accounts for almost 30% to 45% of all genotyped congenital LQTS cases. Approximately 200 mutations in this gene have been identified, which result in rapid closure of the hERG channels and decrease the normal rise in I Kr , leading to delayed ventricular repolarization and QT prolongation. Mutations involving the pore region of the hERG channel are associated with a significantly more severe clinical course than nonpore mutations; most pore mutations are missense mutations with a dominant-negative effect.
LQT6 is caused by loss-of-function mutations of the KCNE2 gene, which encodes the accessory β-subunit (MiRP1) of the hERG channel. LQT6 displays clinical resemblance to LQT2.
Mutations related to the inward rectifier potassium current.
Inward rectifier potassium current (I K1 ) contributes to the terminal portion of phase 3 repolarization. Andersen-Tawil syndrome (LQT7) is caused by loss-of-function mutations of the KCNJ2 gene, which encodes the voltage-dependent K + channel (Kir2.1) that contributes to the inward I K1 . Kir2.1 channels are expressed primarily in skeletal muscle, heart, and brain. The majority of mutations exert a dominant-negative effect on channel current.
Disruption of the I K1 function can potentially lead to prolongation of the terminal repolarization phase and QT interval, which can predispose to the generation of early afterdepolarizations (EADs) and delayed afterdepolarizations (DADs) causing ventricular arrhythmias. However, unlike other types of LQTS, where the afterdepolarizations arise from reactivation of L-type Ca 2+ channels, the EADs/DADs generated in LQT7 are likely secondary to Na + -Ca 2+ exchanger-driven depolarization. It is believed that the differential origin of the triggering beat is responsible for the observed discrepancy in arrhythmogenesis and the clinical features compared with other types of LQTS. In addition, it is likely that prolongation of the action potential duration in LQT7 is somewhat homogeneous across the ventricular wall (i.e., transmural dispersion of repolarization is less prominent than in other types of LQTS), which can potentially explain the low frequency of torsades de pointes. Flaccid paralysis results from failure to propagate action potentials in the skeletal muscle membrane as a result of sustained membrane depolarization.
Mutations related to the acetylcholine-activated potassium current.
LQT13 is caused by loss-of-function mutations of the KCNJ5 gene, which encodes the α subunit (Kir3.4, GIRK4) of the inward acetylcholine-activated potassium current (I KACh ). Kir3.4 mutations exert dominant-negative effects on Kir3.1/Kir3.4 channel complexes by disrupting membrane targeting and stability of Kir3.4.
Mutations Related to the Sodium Current
LQT3 is caused by gain-of-function mutations of the SCN5A gene, which encodes the α subunit (Na v 1.5) of the cardiac voltage-gated Na + channel that is responsible for the sodium content (I Na ). LQT3 accounts for approximately 5% to 10% of genotype-positive LQTS cases. More than 200 mutations have been identified in the SCN5A gene, with the majority being missense mutations mainly clustered in Na v 1.5 regions that are involved in fast inactivation, or in regions that stabilize fast inactivation.
Several mechanisms have been identified to underlie ionic effects of SCN5A mutations in LQT3. Most of the SCN5A mutations cause a gain of function through disruption of fast inactivation of the Na + channel, allowing repeated reopening during sustained depolarization and resulting in an abnormal, small but functionally important sustained (or persistent) noninactivating Na + current (I sus ) during the action potential plateau that acts to slow repolarization and prolong the action potential duration ( eFig. 31.1 ). Other, less common mechanisms ( eFig. 31.2 ) include increased window current, which results from delayed inactivation of mutant Na + channels, occurring at more positive potentials and widening the voltage range during which the Na + channel may reactivate without inactivation. In addition, some mutations cause slower inactivation, which allows longer channel openings, and causes a slowly inactivating Na + current (the late Na + current, I NaL ).


Regardless of the mechanism, increased Na + current (I sus , window current, I NaL , or peak I Na ) upsets the balance between depolarizing and repolarizing currents in favor of depolarization. Gain-of-function LQT3 mutations often increase I NaL two- to fourfold. Because the general membrane conductance is small during the action potential plateau, the presence of a persistent inward Na + current, even of small amplitude, can potentially have a major impact on the plateau duration and can be sufficient to prolong repolarization and QT interval. The resulting delay in the repolarization process triggers EADs (i.e., reactivation of the L-type Ca 2+ channel during phase 2 or 3 of the action potential), especially in Purkinje fiber myocytes where action potential durations are intrinsically longer. Also, increased influx of Na + (via the enhanced I NaL ) can stimulate the Na + -Ca 2+ exchanger in the reverse mode (3 Na + ions out, one Ca 2+ ion in), with consequent intracellular Ca 2+ overload and DADs. QT prolongation and the risk of developing arrhythmia is more pronounced at slow heart rates, when the action potential duration is longer, allowing more Na + current to enter the cell.
LQT9 is caused by gain-of-function mutations of the CAV3 gene, which encodes caveolin-3, a plasma membrane scaffolding protein that interacts with Na v 1.5 and plays a role in compartmentalization and regulation of channel function. Mutations in caveolin-3 induce kinetic alterations of the Na+ channel that result in persistent late Na + current (I sus ) and have been reported in cases of SIDS.
LQT10 is caused by gain-of-function mutations of the SCN4B gene, which encodes the β-subunit (Na v β4) of the Na v 1.5 ion channel. To date, only a single mutation in one patient has been described, which resulted in a shift in the inactivation of the Na + current toward more positive potentials, but did not change the activation. This resulted in increased window currents at membrane potentials corresponding to phase 3 of the action potential.
LQT12 is caused by mutations of the SNTA1 gene, which encodes α1-syntrophin, a cytoplasmic adaptor protein that enables the interaction between Na v 1.5, nitric oxide synthase, and sarcolemmal Ca 2+ adenosine triphosphatase (ATPase) complex that appears to regulate ion channel function. By disrupting the interaction between Na v 1.5 and sarcolemmal calcium ATPase complex, SNTA1 mutations cause increased Na v 1.5 nitrosylation with consequent reduction of channel inactivation and increased I sus densities.
Mutations Related to the Calcium Current
Mutations related to the L-type calcium current.
Timothy syndrome (LQT8) is caused by gain-of-function mutations of the CACNA1C gene, which encodes the α subunit (Ca v 1.2) of the voltage-dependent L-type Ca 2+ channel that contributes to L-type calcium current (I CaL ).
Recently, multiple nonsyndromic LQTS-causative mutations in CACNA1C were described in LQTS patients with isolated QT prolongation and propensity to ventricular arrhythmias in the absence of congenital heart defects and extracardiac manifestations that define Timothy syndrome clinically (nonsyndromic LQT8). Other CACNA1C mutations were found to cause cardiac-only Timothy syndrome (COTS), characterized by the concomitant but variably expressed phenotypes of LQTS (QT prolongation), hypertrophic cardiomyopathy, congenital heart defects, and SCD in the absence of any extracardiac symptoms.
The inward I CaL sustains depolarization and gives rise to the plateau phase essential for excitation-contraction coupling. Gain-of-function mutations of CACNA1C result in near complete elimination of voltage-dependent inactivation of Ca v 1.2 channels, leading to inappropriate continuation of the depolarizing I CaL and lengthening of the plateau phase, predisposing to the generation of EADs. In addition, augmentation of I CaL leads to intracellular Ca 2+ overload, which promotes spontaneous ectopic Ca 2+ release from sarcoplasmic reticulum and the generation of potentially arrhythmogenic DADs.
The exact pathophysiology of hypertrophic cardiomyopathy, congenital heart defects, and extracardiac manifestations of Timothy syndrome and their relation to CACNA1C mutations remain unclear.
Mutations related to calmodulin (calmodulinopathy).
Calmodulin is a small cytoplasmic Ca 2+ -binding protein with ubiquitous expression. Calmodulin is involved, directly and indirectly, in modulation of several ion channels, including Na + , K + , L-type Ca 2+ , and RYR2 channels. The main binding partner of calmodulin in cardiac cells is RyR2, which regulates Ca 2+ release from the sarcoplasmic reticulum. Acting as an intracellular Ca 2+ sensor, Ca 2+ -saturated calmodulin binds RYR2 to inhibit Ca 2+ release by stabilizing the RyR2 channel closed state.
Three different genes (CALM1–3) in the human genome encode exactly the same calmodulin protein. Recent genetic studies have identified several calmodulin mutations associated with CPVT, LQTS, and idiopathic VF. Defective calmodulin–RyR2 binding results in impaired calmodulin inhibition of RyR2 function with consequent dysregulation of sarcoplasmic reticulum Ca 2+ release. Further, de novo mutations in CALM1, CALM2, or CALM3 genes were found to disrupt Ca 2+ -dependent inactivation of the cardiac L-type Ca 2+ channel (Ca v 1.2), which leads in augmentation of I CaL , prolongation of the plateau phase of action potential, and LQTS phenotype (LQT14–16).
Mutations related to triadin: triadin knockout syndrome.
TRDN encodes triadin, a sarcoplasmic reticulum protein functionally and physically related to the RYR2. Homozygous or compound heterozygous loss-of-function mutations in TRDN likely reduce triadin-mediated negative feedback on the L-type Ca 2+ channel, resulting in augmentation of I CaL , prolonged action potential duration, and the recessively inherited LQTS phenotype (LQT17). Intracellular Ca 2+ overload and increases in spontaneous sarcoplasmic reticulum Ca 2+ release, particularly in the setting of beta-adrenergic stimulation, result in ventricular arrhythmias.
LQT17 is characterized by extensive T wave inversions in the precordial leads V 1 through V 4 , with either persistent or transient QT prolongation, exercise-induced cardiac arrest in early childhood (2 to 6 years of age), and mild-to-moderate proximal skeletal muscle weakness. Because all TRDN -null patients display a strikingly similar phenotype, it has been proposed that either triadin knockout syndrome or TRDN -mediated autosomal-recessive LQTS should be used rather than LQT17.
Mutations in the Ankyrin-B Gene: Ankyrin-B Syndrome
LQT4 is caused by loss-of-function mutations of the ANK2 gene, which encodes ankyrin-B, a structural membrane adapter protein that anchors ion channels to specific domains in the plasma membrane. Functionally, ankyrins bind to several ion channel proteins, targeting these proteins to specialized membrane domains, such as the anion exchanger (chloride-bicarbonate exchanger), Na + -K + ATPase, I Na , the Na + -Ca 2+ exchanger (I Na – Ca ), and Ca 2+ release channels (including those mediated by the receptors for inositol triphosphate [IP 3 ] or RyR2). Hence, ANK2 mutations can potentially result in improper localization and activity of ion-conducting proteins.
Mutations of the ANK2 gene have been reported to lead to increased intracellular concentration of Ca 2+ and, sometimes, fatal arrhythmia. However, QT interval prolongation is not a consistent feature in patients with ankyrin-B dysfunction, and the clinical phenotypes often extend beyond the typical LQTS, including sinus node dysfunction (SND), atrioventricular (AV) block, and atrial fibrillation (AF), in addition to idiopathic VF, exercise-induced, polymorphic VT, and SCD. Therefore ankyrin-B dysfunction is now regarded as a clinical entity distinct from classic LQTS (referred to as the “ankyrin-B syndrome”).
Pathophysiology of the Long QT Syndrome
Mechanism of QT Interval Prolongation
Any factor that evokes lengthening of the action potential duration holds the potential of causing an LQTS phenotype, especially if it does it heterogeneously. Electrophysiologically, prolongation of the action potential duration and QT interval can arise from either a decrease in the outward repolarizing current (K + currents: I Kr , I Ks , I K1 , I KACh ) or an increase in inward depolarizing membrane current (I Na , I CaL ) during phases 2 and 3 of the action potentials ( Fig. 31.2 ).

Most commonly, QTc prolongation is produced by delayed repolarization due to attenuation of I Ks (LQT1, LQT5, LQT11), I Kr (LQT2, LQT6), I K1 (LQT7), or I KACh (LQT13). Less commonly, QT prolongation results from prolonged depolarization due to an increase in I Na (LQT3, LQT4, LQT9, LQT10, LQT12) or I CaL (LQT8, LQT14–17).
Mechanism of Dispersion of Repolarization
LQTS is caused by an excessive and heterogeneous prolongation of the repolarization phase of the ventricular action potential. In the normal ventricle, there are heterogeneous cell types with different action potential morphologies and durations, mainly attributed to cell-specific and regional variability in the functional expression of different populations of ion channels (transient outward K + channels [I to ], I Ks ) and the Na + window current (I Na ), and their accessory proteins. Some experimental studies proposed the presence of three irregular cell layers in the ventricular wall with distinct electrical properties: endocardial, midmyocardial (putative M cells), and epicardial cells. Overall, the midmyocardial cells (which have a smaller I Ks , a larger late I Na , and a larger Na + -Ca 2+ exchange current [I Na – Ca ]) appear to generate longer action potential durations that are more susceptible to modification compared with the endocardium and epicardium. The epicardial cells have the shortest action potential durations because of a prominent I to . Repolarization of endocardial cells usually occurs between repolarization of the epicardial and midmyocardial cells. Notably, factors that prolong the action potential appear to elicit a disproportionate prolongation of the action potential duration in midmyocardial cells. As a result, dispersion of the action potential duration becomes irregularly exaggerated across the ventricular wall, yielding an increase in the action potential duration heterogeneity.
Conditions leading to a reduction in I Kr (e.g., LQT2) or augmentation of late I Na (e.g., LQT3) produce a preferential prolongation of the midmyocardial cell action potential. Consequently, QT interval prolongation is accompanied by a dramatic increase in transmural dispersion of repolarization. In contrast, conditions leading to a reduction in I Ks alone (e.g., LQT1) result in a homogeneous prolongation of action potential duration across the ventricular wall with little increase in transmural dispersion of repolarization. However, concurrent beta-adrenergic stimulation (e.g., exercise, isoproterenol) results in abbreviation of epicardial and endocardial action potential duration with little or no change in the midmyocardial cell action potential, resulting in marked augmentation of transmural dispersion of repolarization and arrhythmogenesis (see Fig. 31.2 ).
Mechanism of Torsades de Pointes
The excessive increase in the spatial and/or temporal heterogeneity of the duration of the action potential favors the generation of EADs caused by reactivation of L-type Ca 2+ channels, and on some occasions the late I Na or Na + -Ca 2+ exchanger. EADs can cause premature ventricular complexes (PVCs) (predominantly arising from the Purkinje network) that can potentially infringe on the underlying substrate of heterogeneous repolarization to initiate polymorphic reentrant VT. Excessive transmural heterogeneity of the action potential duration provides the substrate for unidirectional block and functional reentry circuits to perpetuate torsades de pointes. Although controversy still exists, it is likely that the propagation of torsades de pointes is facilitated by intramural reentrant mechanisms giving rise to one or more intramural rotors that impose a rapid ectopic ventricular rhythm (typically 150 to 300 beats/min) while migrating inside the ventricular wall. Hence, with each new cycle the principal depolarization focus migrates accordingly, resulting in a progressive change of the electrical axis, typically rotating 180 degrees in approximately 10 to 12 cycles. This results in a polymorphic VT with the characteristic sinusoidal “twisting of the points” pattern on the 12-lead electrocardiogram (ECG).
Notably, although the atrium seems to be resistant to generating EADs in response to agents that prolong repolarization, atrial EADs and “atrial torsades de pointes” have been reported in some LQTS patients as well as cesium-treated dogs.
Mechanism of Exercise-Induced QT Interval Changes
Arrhythmic events in LQTS are strongly associated with triggers linked to inappropriate QT adaptation to changes in heart rate. Patients with LQT1 and LQT2 genotypes have differing patterns of QT adaptation during beta-adrenergic stimulation (e.g., during stress, exercise, or epinephrine infusion). LQT1 patients appear to have less repolarization reserve during exercise as evidenced by a progressive or persistent pattern of QTc prolongation at faster heart rates, compared with patients with LQT2, in whom maximal QTc prolongation occurs at submaximal heart rates in the early phase of sympathetic stimulation with subsequent fall toward baseline values at faster heart rates.
I Ks is an important determinant of rate-dependent shortening of the cardiac action potential and QT interval. As heart rate increases, I Ks increases because channel deactivation is slow and incomplete during the shortened diastole. This allows I Ks channels to accumulate in the open state during rapid heart rates and contribute to the faster rate of repolarization. Furthermore, I Ks is markedly enhanced by beta-adrenergic stimulation through G-protein/cAMP-mediated channel phosphorylation by protein kinase A (PKA) (requiring AKAP9 [Yotiao]) and PKC (requiring MinK). Importantly, I Ks is functionally upregulated when other repolarizing currents (such as I Kr ) are reduced, potentially serving as a “repolarization reserve” and a safeguard against loss of repolarizing power, especially when beta-adrenergic stimulation is present. LQT1 subjects have compromised I Ks channels that are not as responsive to sympathetic stimulation, and phase 3 repolarization in these individuals is retarded. Consequently, during beta-adrenergic stimulation, there are relatively more unopposed depolarizing forces via the L-type Ca 2+ channel and the Na + -Ca 2+ exchanger that prolong the action potential duration and the QT interval.
In contrast, subjects with LQT2 have dysfunctional I Kr channels, which represent a smaller fraction of the K + channels responsible for phase 3 repolarization and are not as sympathetically responsive as I Ks channels. Therefore, in LQT2 patients, the QT fails to shorten at the intermediate heart rates in the early phase of exercise or epinephrine infusion because of attenuation of I Kr . This is followed by recruitment of I Ks (“repolarization reserve”) at faster heart rates during continuing exercise or epinephrine infusion, with concomitant appropriate abbreviation of the action potential duration and QT shortening, which persists into the recovery phase. This consequently leads to an exaggerated QT difference between the exercise and recovery QT/R-R curves that is manifested as increased QT hysteresis, which appears to be a characteristic feature of the LQT2 phenotype.
The LQT3 phenotype is characterized by a constant reduction of the action potential duration with epinephrine because of stimulation of the intact I Ks channel and augmentation of the late inward I Na . In fact, LQT3 patients may have supernormal QT adaptation in response to exercise compared with control subjects. SCN5A mutations in LQT3 cause a gain of function through disruption of fast channel inactivation, allowing repeated reopening during sustained depolarization and resulting in a small but functionally important enhancement of the I Na during action potential plateau. As a consequence, the risk of developing arrhythmia will be expected to be particularly high at slow heart rates, when the action potential duration is longer, allowing more Na + current to enter the cell.
The differences in the dynamic response of ventricular repolarization to sympathetic stimulation may explain the epidemiological observation that patients with LQT1 are more likely to have life-threatening events during sympathetic activation compared with patients with other genotypes and also may underlie the responsiveness of LQT1 patients to beta-blocker therapy.
Mechanism of Genotype-Phenotype Variability
The LQTS is a complex and multifactorial disorder characterized by significant phenotypic heterogeneity. The clinical phenotype (QTc values, arrhythmia-related symptoms, and outcomes) is highly variable, with a broad continuous spectrum of clinical or subclinical phenotypes, not only between families carrying different pathogenic mutations, but also among family members carrying an identical mutation. One end of this spectrum is concealed LQTS (silent carriers of disease-causing mutations), whereby no QT prolongation or related symptoms are observed. At the other end of the spectrum are the severe symptomatic LQTS patients, who often represent the index cases easily identified in families. In between are patients with different degrees of QT prolongation and different levels of severity of arrhythmias.
A multitude of genetic and acquired interacting factors (some defined but many still unknown) influence the pathophysiology and clinical course of each LQTS subject and ultimately determine a spectrum of phenotypes. Among these factors is the fact that action potential generation is a polygenic process; different LQTS genes affect different ion current mechanisms. Even mutations in the same gene can affect gene expression levels and ionic current activity to different extents and via different mechanisms. As noted above, mutations located in the transmembrane segment (for LQT1) or pore region (for LQT2) of the ion channel generally result in more malignant disease compared with mutations in other locations. Similarly, mutations causing a dominant-negative effect (e.g., missense mutations involving the pore region of the channel) result in more profound channel dysfunction and more severe clinical disease than those associated with haplotype insufficiency (e.g., mutations causing coassembly or trafficking abnormalities).
The “repolarization reserve” concept can underlie, at least in part, the phenotypic heterogeneity in LQTS. Cardiac muscle repolarization has built-in redundancy, or reserve, such that perturbation of one ion current does not necessarily result in excessive repolarization changes because other currents can compensate. Hence, there exists an inherent variability of arrhythmic response to QT interval prolongation with the relationship between perturbations of one ion channel in relation to the sum total of repolarizing currents. This concept implies that often multiple “hits” to repolarization are required to compromise repolarization and surpass the threshold for developing clinical QT prolongation and torsades de pointes. In this setting, a mutation in one of the LQT-linked genes causing an attenuation of a cardiac ionic current may result in only a limited disruption of the repolarization process, which can be clinically concealed and become unmasked (manifesting as QT prolongation and arrhythmias) only when accompanied by another insult to the same or a different ionic current (e.g., drugs or electrolyte abnormalities). In fact, it has been suggested that some cases of acquired LQTS represent inadvertent “unmasking” of subclinical congenital LQTS.
Adding to the complexity is the “double-hit” phenomenon, secondary to either two mutations in the same gene (compound heterozygosity) or mutations in two different LQT genes (digenic heterozygosity). Double hits occur in 5% to 10% of LQTS patients and the resulting phenotype is more severe than with a single hit.
Genetic factors are also involved in the control of cardiac repolarization at the population level. The heritability of the QTc interval has been estimated as being between 25% and 52%. Ventricular action potential is under the joint control of multiple ionic currents, and the activity and expression levels of the channels underlying each of these currents establish a subtle equilibrium between depolarizing and repolarizing currents determining the action potential duration in each individual. Common genetic variants differing from the ancestor sequence by one nucleotide (i.e., single nucleotide polymorphism) in genes coding for proteins that are known or suggested to affect ion channel function appear to influence this equilibrium even via weak effects on activity and/or expression level of channel subunits and can potentially play a role in determining cardiac repolarization duration and QTc length in healthy individuals. Therefore apart from the known LQT-linked genetic mutations, allelic variation elsewhere in the genome, most often single nucleotide polymorphisms, in the same disease-causing gene or in other genes, can amplify otherwise subclinical disturbances of the repolarization into overt LQTS and potentially contribute to the variable penetrance and clinical phenotype heterogeneity.
Furthermore, the resultant intrinsic risk for arrhythmias can be modulated by a variety of intrinsic or extrinsic environmental factors, including age, gender, heart rate, sympathetic tone, electrolyte balance, presence of QT-prolonging drugs, as well as inherited and acquired pathological conditions such as LV hypertrophy, and heart failure.
In summary, the interaction of the underlying LQTS genetic mutation with other genetic factors in the same gene or elsewhere in the host genome (“modifier genes”), as well as with multiple superimposed acquired risk determinants (“disease modifiers”), has a substantial impact on the expressivity of the phenotype of the LQT genotype.
Mechanism of Gender Effects
In the healthy population, QTc intervals are longer in women than in men, a difference that becomes apparent only after puberty. This is associated with a higher susceptibility to drug-induced LQTS and torsades de pointes; women account for 70% of cases of drug-induced QT prolongation and torsades de pointes. In healthy volunteers, drug-induced QT interval prolongation is greatest during the menses and ovulation phases and least during the luteal phase. Age-dependent gender-related differences also exist among patients with congenital LQTS. Although the risk of cardiac events is higher in boys with LQTS as compared with girls during childhood and early adolescence, a gender risk reversal occurs after the onset of puberty. LQT1 and LQT2 women of childbearing age have longer QT intervals and higher risk for polymorphic VT and SCD than men. The extent of QT prolongation and the risk of cardiac events appear to be more pronounced during periods with high estradiol and low progesterone levels (e.g., during the follicular phase of the menstrual cycle) and mildly reduced during periods with higher progesterone/estradiol ratio (e.g., during the luteal phase of the menstrual cycle, and during pregnancy). The arrhythmogenic risk markedly increases immediately postpartum (especially among women with the LQT2 genotype), when serum progesterone concentrations abruptly decline.
The mechanisms underlying these gender-related differences are poorly understood. Environmental (increased physical activity), hormonal, and genetic factors (modifier genes not shared by boys and girls) have been proposed. Current evidence supports the role of sex hormones as a significant contributor to the action potential duration. Recent studies suggest that the duration of ventricular repolarization and QTc interval are influenced by complex interactions between sex steroid hormones and gonadotropins depending on gender, rather than on one single hormone. In general, testosterone in men and an increased progesterone/estradiol ratio in women shorten repolarization, whereas follicle-stimulating hormone prolongs repolarization in both genders.
The effect of sex hormones on the QT interval and arrhythmogenesis in LQTS can partly be explained by direct and indirect interaction with the ion channel. Experimental studies suggest that estrogen suppresses I Kr , and increases I CaL , Na + -Ca 2+ exchanger activity, RyR2 leakiness, and alpha 1 – and beta 2 -adrenoceptor responsiveness. Estradiol also enhances the sensitivity of I Kr channels to their specific antagonist and predisposes to greater drug-induced QT prolongation. Conversely, testosterone enhances the outward currents (I Kr , I Ks , I K1 ) and reduces the inward current (I CaL ). Progesterone, a testosterone precursor, enhances I Ks and reduces I CaL . Thus estradiol can potentially exert a proarrhythmic effect in LQTS patients, whereas testosterone and progesterone shorten QT duration and exert an antiarrhythmic effect with a reduced susceptibility to sympathetic stimuli. In fact, a recent study demonstrated potential benefit of oral progesterone for the prevention of drug-induced QT interval prolongation.
Epidemiology
There are no systematic studies on LQTS prevalence in the general population. A recent estimate of the prevalence of LQTS is 1 : 2000 live births, based on the results of genetic screening in families and the incidence of compound heterozygotes (i.e., persons with two mutations). However, the clinical disease is less common (approximately 1 in 5000) because most mutation carriers remain asymptomatic. The usual mode of inheritance is autosomal dominant, with the exception of the autosomal recessive Jervell and Lange-Nielsen type and triadin knockout syndrome (LQT17).
LQT1, LQT2, and LQT3 comprise more than 90% of all genotyped LQTS cases. LQT1 is the most frequent genetic form of LQTS, accounting for 40% to 55% of genotyped LQTS cases. LQT2 and LQT3 account for 30% to 45% and 5% to 10% of genotyped LQTS cases, respectively ( Table 31.2 ). The remaining 14 types (LQT4 to LQT17) make up less than 5% of the genotype-identified LQTS. Although LQT3 is infrequent among adolescents and adults with LQTS, it constitutes the most common genetic form associated with LQTS-related perinatal and early-infancy mortality.
LQT1 | LQT2 | LQT3 | |
---|---|---|---|
Pathophysiology | |||
Gene | KCNQ1 (Kv LQT1) | KCNH2 (HERG) | SCN5A |
Protein | K v 7.1 | K v 11.1 | Na v 1.5 |
Ionic current | Decreased I Ks | Decreased I Kr | Increased late I Na |
Clinical Presentation | |||
Incidence of cardiac events | 63% | 46% | 18% |
Incidence of SCD | 4% | 4% | 4% |
Arrhythmia triggers | Emotional/physical stress (swimming, diving) | Emotional stress, arousal (alarm clock, telephone), rest | Sleep/rest |
Electrocardiogram | Broad-based T wave | Low-amplitude, bifid T wave | Long isoelectric ST segment |
QT response to exercise | Attenuated QTc shortening and an exaggerated QTc prolongation during early and peak exercise | Normal QT during exercise but with exaggerated QT hysteresis | Supernormal QT shortening |
Management | |||
Exercise restriction | +++ | ++ | ? |
Response to beta-blockers | +++ | +++ | ? |
Potassium supplement | + | ++ | + |
Left cervicothoracic sympathectomy | ++ | ++ | ++ |
Response to mexiletine | + | + | ++ |
LQTS exhibits incomplete penetrance (i.e., not all carriers of the pathogenic mutation will develop a phenotypic expression) and variable expressivity (i.e., the level of phenotypic expression varies among affected patients).
Clinical Presentation and Natural Course
Romano-Ward Syndrome
Most information regarding the clinical features of LQTS have been derived from analysis of data from large series of LQTS patients, the largest of which is the International LQTS Registry. LQTS probands are diagnosed at an average age of 21 years. The clinical course of patients with LQTS is variable, and is influenced by age, gender, genotype, environmental factors, therapy, and possibly other modifier genes. At least 37% of individuals with the LQT1 phenotype, 54% with the LQT2 phenotype, and 82% with the LQT3 phenotype remain asymptomatic and many are referred for evaluation because of the diagnosis of LQTS of a family member or the identification of a long QT interval on a surface ECG obtained for unrelated reasons.
Symptomatic patients can present with palpitations, presyncope, syncope, or cardiac arrest. Syncope is the most frequent symptom, occurring in 50% of symptomatic probands by the age of 12 years, and in 90% by the age of 40 years. The incidence of syncope in LQTS patients is approximately 5% per year, but can vary depending on the LQTS genotype. Syncope in patients with LQTS is generally attributed to polymorphic VT (torsades de pointes), but can also be precipitated by severe bradycardia in some patients with LQT3. Cardiac arrest and SCD are usually due to VF. Recurrent syncope can mimic primary seizure disorders.
The incidence of SCD is much lower, approximately 1.9% per year. Nonfatal events (syncope and aborted cardiac arrest) in LQTS patients remain the strongest predictors of subsequent LQTS-related fatal events. The overall risk of subsequent SCD in an LQTS patient who has experienced a previous episode of syncope is approximately 5% per year. Of individuals who die of complications of LQTS, SCD is the first sign of the disorder in an estimated 10% to 15%. The risk for SCD from birth to age 40 years has been reported as approximately 4% in each of the phenotypes.
Risk and lethality of cardiac events among untreated individuals are strongly influenced by the genotype. The frequency of cardiac events is significantly higher among LQT1 (63%) and LQT2 (46%) patients than among patients with the LQT3 genotype (18%). However, the likelihood of dying during a cardiac event is significantly higher among LQT3 patients (20%) than among those with the LQT1 (4%) or the LQT2 (4%) genotype. Overall, LQT3 is to be considered a severe LQTS variant; the first cardiac event is more likely to be lethal and seems to occur later in childhood, during or after puberty.
Cardiac events (syncope, cardiac arrest, SCD) in LQTS patients do not occur at random; the factors precipitating cardiac events seem to be specific for each genetic variant. LQT1 patients present an increased risk during physical or emotional stress (90%), and only 3% of the arrhythmic episodes occur during rest or sleep. Swimming and diving appear as highly specific triggers in LQT1 patients. LQT2 patients are at higher risk for lethal events during arousal (44%), but are also at risk during sleep and at rest (43%). Only 13% of cardiac events occur during exercise. Cardiac events in LQT2 patients are characteristically associated with arousal and auditory stimulation. In fact, the triggering of events by startling, sudden awakening, or sudden loud noises (such as a telephone or alarm clock ring) is virtually diagnostic of LQT2. Notably, individual factors such as gender, location and type of mutation, and QTc prolongation appear to be associated with trigger-specific events; female adolescents with LQT2 appear to experience a greater than ninefold increase in the risk for arousal-triggered cardiac events compared with male adolescents in the same age group. In contrast, gender does not seem to be a significant risk factor for exercise-triggered events among carriers of the same genotype ( Fig. 31.3 ).

On the other hand, LQT3 patients experience cardiac events largely while asleep or at rest (65%) without emotional arousal, and only occasionally during exercise (4%). Notably, the majority of patients continue to experience their cardiac events under conditions similar to their first classified event.
The effect of gender on outcome is age dependent, with boys being at higher risk than girls during childhood and early adolescence, but no significant difference in gender-related risk being observed between 13 and 20 years. The gender-related risk reverses afterward, and female patients maintain higher risk than male patients throughout adulthood.
The genotype can potentially affect the clinical course of the LQTS and modulate the effects of age and gender on clinical manifestations. Although the three major LQTS genotypes (LQT1, LQT2, or LQT3) are associated with similar risks for life-threatening cardiac events in children and adolescents after adjustment for clinical risk factors (including gender, QTc duration, and time-dependent syncope), the risk for cardiac events is augmented in LQT2 women aged 21 to 40 years and in LQT3 patients greater than 40 years of age. The risk of syncope and SCD decreases during pregnancy but increases in the postpartum period, especially among LQT2 women.
Jervell and Lange-Nielsen Syndrome
The Jervell and Lange-Nielsen syndrome is the recessive variant of the LQTS, characterized by congenital deafness and cardiac phenotype (QT prolongation, ventricular arrhythmias, and SCD). The Jervell and Lange-Nielsen syndrome is caused by two homozygous or compound heterozygous mutations of one of the two genes ( KCNQ1 and KCNE1 ) that encode components of the I Ks channel.
Patients with Jervell and Lange-Nielsen syndrome have a more severe cardiac phenotype than those with Romano-Ward syndrome. Patients begin to experience cardiac events very early in life; 15% suffer a cardiac event during the first year of life, 50% by age 3 years, and 90% by age 18 years. In untreated patients, approximately 50% die of ventricular arrhythmias by the age of 15 years. Furthermore, SCD occurs in more than 25% of patients despite medical therapy. In addition, complete loss of I Ks in hair cells and endolymph of the inner ear results in congenital bilateral sensorineural deafness.
The conditions that trigger the cardiac events are, overall, very similar to those described for LQT1. Up to 95% of events occur during sympathetic activation (exercise and emotions), and only 5% of the events occur at rest or during sleep.
Andersen-Tawil Syndrome
Andersen-Tawil syndrome (LQT7) is a rare autosomal dominant disorder caused by mutations of the gene KCNJ2, which encodes the inward rectifier potassium channel, Kir2.1. This syndrome is characterized by a triad of a cardiac phenotype, a skeletal muscle phenotype (periodic paralysis caused by abnormal muscle relaxation), and distinctive craniofacial and skeletal dysmorphic features (low-set ears, ocular hypertelorism, small mandible, fifth-digit clinodactyly, syndactyly, short stature, scoliosis, and a broad forehead).
Although Andersen-Tawil syndrome is classified as LQTS (LQT7), QT prolongation is more related to a prominent U wave (i.e., prolonged “QTUc interval”) rather than a QTc prolongation. Further, unlike other types of LQTS, cardiac arrhythmias in Andersen-Tawil syndrome are characterized by high burden of asymptomatic PVCs, ventricular bigeminy, or nonsustained VT, with a predominantly benign course and very rare degeneration into hemodynamically compromising rhythms like torsades de pointes. In addition, a specific type of polymorphic VT, bidirectional VT, is observed in Andersen-Tawil syndrome, similar to that observed in CPVT patients. Beta-blockers, Ca 2+ channel blockers, and flecainide have been utilized for suppression of ventricular arrhythmias in these patients, but the efficacy of these drugs is yet to be proven.
Patients with Andersen-Tawil syndrome present initially with periodic paralysis or cardiac symptoms (palpitations, syncope, or both) in the first or second decade of life. Intermittent weakness occurs spontaneously, or can be triggered by prolonged rest or rest following exertion; however, the frequency, duration, and severity of symptoms are variable between and within affected individuals, and are often linked to fluctuations in plasma K + levels. Mild permanent weakness is common.
There is a high degree of variability in penetrance and phenotypic expression. Approximately 60% of affected individuals manifest the complete triad and up to 80% express two of the three cardinal features.
Timothy Syndrome
Timothy syndrome (LQT8) is an extremely rare multisystem disorder caused by mutations of the CACNA1C gene, which encodes the L-type Ca 2+ channel (Ca v 1.2), and is characterized by syndactyly, QT prolongation, congenital heart disease, cognitive and behavioral problems, musculoskeletal diseases, immune dysfunction, and more sporadically, autism.
Timothy syndrome is a severe form of LQTS, characterized by a remarkable prolongation of the QTc interval (QTc often exceeding 550 to 600 milliseconds), functional 2 : 1 AV block (observed in up to 85% of patients, and likely caused by the extremely prolonged ventricular repolarization and refractory periods), and macroscopic T wave alternans (positive and negative T waves alternating on a beat-to-beat basis). In addition, congenital heart defects are observed in approximately 60% of patients and include patent ductus arteriosus, patent foramen ovale, ventricular septal defect, tetralogy of Fallot, and hypertrophic cardiomyopathy. Timothy syndrome is highly malignant; the majority of patients seldom survive beyond 3 years of age. Polymorphic VT and VF occur in 80% of patients (commonly triggered by an increase in sympathetic tone) and are the leading cause of death, followed by infection and complications of intractable hypoglycemia.
Extracardiac features include cutaneous syndactyly (variably involving the fingers and toes), which is observed in almost all patients. Facial findings (observed in approximately 85% of individuals) include low-set ears, flat nasal bridge, thin upper lip, small upper jaw, small, misplaced teeth, and round face. Neuropsychiatric involvement occurs in approximately 80% of individuals and includes global developmental delays and autism spectrum disorders.
In general, the diagnosis of Timothy syndrome is made within the first few days of life based on the markedly prolonged QT interval and 2 : 1 AV block. Occasionally, the diagnosis is suspected prenatally because of fetal distress secondary to AV block or bradycardia.
Although LQT8 (Timothy syndrome) is associated with gain-of-function in I CaL , reduction of I CaL influx by Ca 2+ channel blockade (such as verapamil) failed to shorten QTc or prevent ventricular arrhythmias. A recent report suggested a potential antiarrhythmic benefit of ranolazine (an antianginal agent with inhibitory effects on multiple ion channels) in patients with Timothy syndrome.
Electrocardiographic Features
Abnormal prolongation of the QT interval on the surface ECG, reflecting delayed ventricular repolarization, is the hallmark of LQTS. In addition, T wave abnormalities are encountered in the majority of patients.
QT Interval Measurement
The QT interval is the body surface representation of the duration of ventricular depolarization and subsequent repolarization. Any deviation or dispersion of depolarization (e.g., bundle branch block) or repolarization (e.g., prolongation or dispersion of the action potential duration) results in prolongation of the QT interval.
An accurate measurement of the QT interval is important for the diagnosis of LQTS. A 12-lead ECG tracing at a paper speed of 25 mm/s at 10 mm/mV is usually adequate to make accurate measurements of the QT interval. The QT interval is measured as the interval from the onset of the QRS complex, that is, the earliest indication of ventricular depolarization, to the end of the T wave, that is, the latest indication of ventricular repolarization. The QT interval is measured in all ECG leads where the end of the T wave can be clearly defined (preferably leads II and V 5 or V 6 ), with the longest value being used. The end of the T wave is the point at which the descending limb of the T wave intersects the isoelectric line. Three to five consecutive cardiac cycles are taken to derive average values for R-R, QRS, and QT intervals.
When the end of the T wave is indistinct, or if a U wave is superimposed or inseparable from the T wave, it is recommended that the QT be measured in the leads not showing U waves (often leads aVR and aVL) or that the downslope of the T wave be extended by drawing a tangent to the steepest proportion of the downward limb of the T wave until it crosses the baseline (i.e., the T-P segment) ( Fig. 31.4 ). However, it should be recognized that defining the end of the T wave in these ways can potentially underestimate the QT interval. Some investigators advocate measurement of both the QT interval and the QTU interval (with the latter measurement taken to the end of the U wave as it intersects the isoelectric line) because the QTU interval probably reflects the total duration of ventricular depolarization.

The highest diagnostic and prognostic value in LQTS families has been observed for QTc in leads II and V 5 of the 12-lead ECG. Thus QTc should be obtained in one of these leads if measured in only a single ECG lead. However, other leads were found to offer similar diagnostic (aVR) or prognostic (V 2 /V 3 ) value alone, and, in general, the lead with the longest QT interval is used for measurement.
The QT interval should ideally be measured at a heart rate closest to 60 beats/min and definitely less than 100 beats/min. The use of beta-blockers or long-term ECG monitoring need to be considered to obtain optimal measurements.
QT Interval Correction for Gender
The QT interval shortens after puberty in males but not in females, resulting in a longer QT in women than in men. The reported gender difference in various studies varies from 6 to 10 milliseconds in older age groups and from 12 to 15 milliseconds in younger adults. Overall, the gender difference in the QTc interval becomes small after 40 years of age and practically disappears in older men and women. Separate gender- and age-specific QT adjustment formulas have been proposed to accommodate these differences.
QT Interval Correction for Heart Rate
Because the heart rate (R-R cycle length) is the primary modifier of ventricular action potential, QT interval measurements must be corrected (QTc) for the baseline R-R interval to allow for comparisons. Various correction formulas have been developed ( Table 31.3 ), the most widely used being the formula derived by Bazett in 1920 from a graphic plot of measured QT intervals in 39 young subjects. The Bazett correction, however, performs less well at high and low heart rates (undercorrects at fast heart rates and overcorrects at slow heart rates).
Bazett | QTcB = QT/(R-R interval) 1/2 (all intervals in seconds) |
Framingham-Sagie | QTcFa = QT + 154(1 − 60/HR) |
Fridericia | QTcFi = QT/(R-R interval) 1/3 |
Hodges | QTcH = QT + 1.75(HR − 60) |
Nomogram-Karjalainen | QTcN = QT + nomogram correction factor |
Rautaharju | QTcRa = QT − 155 × (60/HR − 1) − 0.93 × (QRS − 139) + k (k = −22 msec for men, and –34 msec for women) |
In addition to the Bazett formula, many other correction formulas, such as the Framingham-Sagie, Fridericia, Hodges, and Nomogram-Karjalainen formulas, have been proposed. In a study comparing the accuracy of those five different QT correction formulas for determining drug-induced QT interval prolongation, the Bazett correction formula provided the most marked QTc variations at heart rates distant from 60 beats/min. The Fridericia formula was found to overestimate QTc at faster heart rates, being more reliable at slower heart rates. Conversely, the Hodges, Nomogram, and Framingham formulas demonstrated less QTc variability over the whole range of the investigated heart rates and seemed to be similarly satisfactory at heart rates of up to 100 beats/min. Among them, the Hodges method, followed by the Nomogram-Karjalainen method, appeared to be the most accurate in determining the correct QTc and subsequently in guiding clinical decisions.
Nonetheless, the Bazett formula remains the one most commonly used to measure the QT interval for the diagnosis of LQTS. However, when a prolonged QTc is observed during faster heart rates (greater than 90 beats/min), it is important to repeat the ECG once the heart rate slows down, to minimize the error that can potentially be introduced by heart rate correction formulas.
Importantly, there exists a substantial interindividual variability of the relationship between QT duration and heart rate (the QT/R-R relationship). In contrast, a high intraindividual stability of the QT/R-R pattern has been demonstrated, suggesting that a genetic component might partly determine individual QT length. Therefore population-based and averaged QT correction cannot accurately predict a normal QT interval at a given R-R interval in a given patient. Individual-specific QT/R-R hysteresis correction in combination with individualized heart rate correction can potentially reduce intraindividual QTc variability.
QT Interval Correction to QRS Duration
The QT interval prolongs in ventricular conduction defects, mainly due to a delay in depolarization (i.e., prolongation of the QRS duration) and not in repolarization. Most QT correction formulae do not take bundle branch block into consideration. Only a few formulae (such as the Rautaharju formula, the Bogossian formula, and the JT index) are designed to include bundle branch blocks in their calculations, but none of them have been widely adopted in clinical practice.
A widely used approach is to apply the Bazett formula to the uncorrected QT interval and accept a longer QTc as the upper limit of acceptable (550 milliseconds rather than 500 milliseconds). Another method to adjust the QT measurement after the development of a bundle branch block is to subtract the difference in QRS widths before and after the block (when such information is available). A third method is to measure the JT interval from the end of the QRS complex to the end of the T wave. Unlike the QTc interval which describes both cardiac depolarization and repolarization, the JTc interval is independent of the QRS duration and thus represents only repolarization (JTc = QTc − QRS). If the JT interval is chosen, normal standards established specifically for the JT interval should be used. QT and JT adjustment formulas have recently been introduced for use in the setting of prolonged ventricular conduction. With confirmation, they may be incorporated into automated algorithms to provide appropriate correction factors. However, because of delay in depolarization in left bundle branch block (LBBB), repolarization begins before the end of the QRS complex (i.e., before the J point); hence, the JT interval likely underestimates the duration of repolarization, particularly when QRS duration was very prolonged (greater than 175 milliseconds).
A recent study in a series of patients with intermittent LBBB found that the net increase in QRS duration contributes to 92% of the extra time in measured QT in LBBB, and a new formula (the Wang formula) was developed to calculate the true QT: True QT interval = measured QT in LBBB − (0.86 * QRS in LBBB − 71). The QT so derived can then be corrected using the Bazett or other standard formulation.
QT Interval Measurement During Right Ventricular Pacing
Reliable estimation of the QT interval in ventricular paced rhythm is challenging. It has been demonstrated that the Rautaharju formula (see Table 31.3 ) yields QTc values during ventricular pacing that are closest with the non-paced QTc interval values; however, this formula is complex to apply and exhibits significant interaction with heart rate changes.
The Framingham and Nomogram correction methods were found to offer the most optimal balance for assessing the QTc interval, including minimal interaction between pacing mode and heart rate. The use of the Bazett formula significantly exaggerates the QTc rate dependency during ventricular pacing and, therefore, is less reliable.
A recent study suggested the use of the Framingham and Nomogram methods to calculate the corresponding nonpaced intrinsic QTc interval from the measured ventricular paced QTc interval (regardless of heart rate) by subtracting 43 milliseconds in normal-QTc patients, and 37 milliseconds (for the Framingham method) or 36 milliseconds (for the Nomogram method), in prolonged-QT patients.
Another study found that RV pacing causes prolongation of the QT due to a paced LBBB without prolongation of the JT interval, and that QT prolongation caused by ventricular pacing constitutes about 50% of the QRS width. The Bogossian formula subtracts this value from the measured ventricular paced QT interval (QT in LBBB = measured QT interval − 50% * QRS duration). Although this formula can provide a simple method for estimating the nonpaced intrinsic QT interval, it tends to overcorrect the QT interval, that is, making the QT shorter than it should be.
The paced JTc interval was found to more accurately represent ventricular repolarization (as compared to the paced QTc interval) and mirror repolarization changes observed during nonpaced rhythm. However, as noted previously, the sensitivity of the JT interval in identifying patients with delayed ventricular repolarization decreases with increasing QRS duration, and that can artificially shorten the calculated QT interval. Therefore the JT interval appears ineffective in predicting delayed repolarization in patients with a very wide QRS.
QT Interval Prolongation
The diagnosis of QT interval prolongation can be challenging because of the difficulty in defining the “end” of the T wave and the need for correction for heart rate, age, and gender. This is further complicated by the lack of linear behavior of the Bazett formula at slower and faster heart rates as well as the arbitrary definition of the gender-based diagnostic cutoff values that define an abnormally prolonged QTc (QTc of 450 milliseconds for men and QTc of 460 milliseconds for women; Table 31.4 ).
Rating | 1–15 Years | Adult Man | Adult Woman |
---|---|---|---|
Normal | <440 | <430 | <450 |
Borderline | 440–460 | 430–450 | 450–470 |
Prolonged | >460 | >450 | >470 |
Furthermore, no single QTc value separates all LQTS patients from healthy controls. In fact, LQTS cannot be excluded merely by the presence of a normal QTc interval. The QT interval is subject to large variations even in healthy individuals, and substantial overlap exists with QTc values obtained from LQTS mutation carriers in the range between 410 and 470 milliseconds. In up to 40% of LQTS patients, QTc intervals fall in the normal range. On the other hand, QTc values greater than 470 milliseconds in men and greater than 480 milliseconds in women are practically not observed among healthy individuals (especially when their heart rate is 60 to 70 beats/min). Therefore unless excessive QTc prolongation (greater than 500 milliseconds, corresponding to the upper quartile among affected genotyped individuals) is present, the QTc interval should always be evaluated in conjunction with the other diagnostic criteria.
Of note, a considerable variability in QTc interval duration can be observed in patients with LQTS when serial ECGs are recorded during follow-up. This time-dependent change in QTc duration is an important determinant of the phenotypic expression of the disease. Up to 40% of patients with LQTS will have QTc greater than 500 milliseconds at least once during long-term follow-up, but only 25% will have that degree of QT prolongation during their initial evaluation. The maximal QTc duration measured at any time before age 10 years was shown to be the most powerful predictor of cardiac events during adolescence, regardless of baseline, mean, or most recent QTc values. In addition, the QT interval may normally exhibit individual variations during the day. Therefore in the case of borderline QTc prolongation, serial ECG and 24-hour Holter recordings can potentially assist in establishing QT prolongation.
T Wave Morphology
ST-T wave abnormalities are common among LQTS patients, and some of the ST-T changes are characteristic for a certain genotype. Specifically, “broad,” “notched,” and “late” T waves are associated with LQT1, LQT2, and LQT3, respectively, although these features are often subtle and inconsistently observed ( Fig. 31.5 ).

Patients with LQT1 commonly exhibit a smooth, broad-based T wave that is present in most leads, and particularly evident in the precordial leads. The T wave generally has a normal to relatively high amplitude and often no distinct onset. LQT2 patients generally present with low-amplitude T waves, which are notched or bifid in approximately 60% of carriers. The bifid T wave can be confused with a T-U complex; however, unlike the U waves, the bifid T waves are usually present in most of the 12 ECG leads. LQT3 patients often show late-onset, narrow, peaked, and/or biphasic T waves with a prolonged isoelectric ST segment. Occasionally, the T wave is peaked and asymmetrical with a steep downslope. These ST-T wave patterns can be seen in 88% of LQT1 and LQT2 carriers and in 65% of LQT3 carriers.
No specific T wave pattern has been suggested in the LQT5 and LQT6 syndromes. T-U wave abnormalities such as biphasic T waves following long pauses like those found in the LQT2 syndrome are commonly observed in the LQT4 syndrome. Enlarged U waves separated from the T wave are reported to be characteristic ECG features in the LQT7 syndrome. Severe QT interval prolongation and macroscopic T wave alternans can be observed in LQT8.
Despite the initial enthusiasm in achieving a genotype-phenotype correlation for specific LQT-associated genes, this approach failed to provide a high diagnostic yield because of the frequent exceptions in T wave morphology presentation. Furthermore, the T wave pattern can vary with time, even in the same patient with a specific mutation. Therefore other T wave parameters, such as duration, amplitude, asymmetry, and flatness, as well as the interval from the peak of the T wave to the end of the T wave (Tp-e), have been used as highly specific quantitative descriptors (see later).
Of note, quantitative analysis of T wave morphology in lead V 6 was found in a recent report to be capable of distinguishing patients with genetically proven LQTS from matched controls and even permits identification of over 80% of patients with LQTS, despite having a normal resting QTc. The potential implications of this tool for universal screening for LQTS warrant further investigation.
Torsades de Pointes
Torsades de pointes is a polymorphic VT occurring in the setting of QT interval prolongation (acquired or congenital LQTS) and is characterized by a progressive change of the electrical axis, typically rotating 180 degrees in approximately 10 to 12 cycles. This results in the characteristic sinusoidal twisting of the peaks of the QRS complexes around the isoelectric line of the recording; hence, the name torsades de pointes or “twisting of the points.” However, this characteristic pattern may not be evident in all ECG leads. Also, during a very fast VT, periodic decrease in the amplitude of the entire QRS-T complex can be observed with less distinct shifts in the QRS axis. Occasionally, a polymorphic QRS configuration is observed without displaying those characteristics. Of note, different patterns can be seen in different VT episodes from the same patient.
The VT is frequently preceded by a variable period of bigeminal rhythm caused by one or two PVCs coupled to the prolonged QT interval of the preceding basic beat. The tachycardia rate is typically in the range of 150 to 300 beats/min. In many cases, torsades de pointes is a self-limiting arrhythmia that spontaneously dies out after a few tens of cycles; however, most patients experience multiple episodes of the VT occurring in rapid succession. Only in a minority of cases does torsades de pointes degenerate into VF, which almost without exception leads to SCD if immediate rescue intervention, including defibrillation, is not performed.
Dispersion of Repolarization
Prolongation of the action potential duration (and QT interval) per se is not pathogenic, as evidenced by the fact that a homogeneous action potential duration prolongation (such as occurs following amiodarone therapy) fails to generate reentry. As demonstrated in experimental models of the LQTS, prolonged repolarization, transmural dispersion of repolarization, and EADs are the three electrophysiological (EP) components linked to the genesis of torsades de pointes. Transmural dispersion of repolarization arises from repolarization heterogeneity that exists between the epicardial and putative midmyocardial (M) cells that lie toward the endocardium of the LV wall. These midmyocardial cells are especially sensitive to a repolarization challenge and exhibit significant prolongation of the action potential duration compared with other transmural cell types. Several ECG indices have been proposed in recent years as noninvasive surrogates for transmural dispersion of repolarization, including the T wave peak to T wave end (Tp-e) interval, QT interval dispersion, and ratio of the amplitudes of the U and T waves.
Tp-e interval.
Although controversy exists, some experimental models suggest that the peak of the T wave coincides with the end of epicardial repolarization (the shortest action potentials), whereas the end of the T wave coincides with the end of repolarization of the M cells (the longest action potentials). Hence, the Tp-e interval in each surface ECG lead has been proposed as an index of transmural repolarization. However, subsequent investigations using intact hearts have challenged this concept and suggested that, in vivo, the Tp-e interval is a measure of “global” dispersion of repolarization of the whole ventricle rather than “transmural” dispersion.
Because increased dispersion of repolarization promotes reentry and enhances arrhythmogenesis, prolongation of the Tp-e interval has been proposed as a more sensitive predictor of risk for arrhythmic events than the QT interval; the latter represents the total duration of electrical ventricular activation and not necessarily the dispersion of transmural repolarization. In fact, a prolonged Tp-e interval has been shown to be a marker of increased arrhythmogenic risk in patients with Brugada syndrome, hypertrophic cardiomyopathy, and structural heart disease, as well as in certain populations.
Although changes in the Tp-e interval can potentially show the dynamicity of the dispersion of repolarization in clinical settings in LQTS patients, the role of measuring the Tp-e interval in these patients has yet to be clearly defined. In fact, the normal value of the Tp-e interval on the ECG has not been established. Nonetheless, an interval of more than 100 milliseconds is uncommon in normal subjects compared with that in patients with LQTS (9% vs. 55%).
On ambulatory monitoring, LQT2 exhibits a larger-degree of dispersion of repolarization, as measured by the Tp-e interval, compared with LQT1 and normal hearts. In fact, the Tp-e interval has been proposed as a diagnostic criterion in differentiation between LQT2 and LQT1 patients. Also, LQT1 patients exhibit abrupt increases in Tp-e intervals at elevated heart rates, whereas LQT2 patients exhibit increases in dispersion of repolarization at a much wider range of rates. In addition, beta-adrenergic stimulation (exercise or epinephrine infusion) increases dispersion of repolarization in both LQTS models, transiently in LQT2 and persistently in LQT1.
QT interval dispersion.
An alternate approach to determine repolarization heterogeneity is provided by the dispersion of the QT interval. The QT dispersion index is obtained by the difference between the maximal and minimal QT intervals (QTmax − QTmin) measured on a 12-lead ECG. The QT dispersion index reflects the spatial heterogeneity of myocardial refractoriness more accurately than single QT values. Visualization of the differences in QT interval in the different ECG leads can be facilitated by the display of temporally aligned simultaneous ECG leads with a slight separation on the amplitude scale.
Importantly, QT interval dispersion measurements are subject to similar shortcomings encountered with the QT interval assessment, as a large overlap between affected and healthy individuals is observed. Further, accurate measurement on the scalar ECG at a paper speed of 25 mm/s is difficult. The same caveat applies to measuring the Tp-e interval.
U wave–to–T wave amplitude ratio.
The ratio of the amplitudes of the U and T waves has been suggested as the clinical counterpart of EADs, and a progressive increase in this ratio was found to precede the onset of torsades de pointes in an experimental model of LQTS. In addition, the increment in U wave amplitude after a PVC has been suggested as a marker for arrhythmia risk in “pause-dependent” LQTS.
In patients with bifid T waves, some investigators used the late component of the T wave, rather than the U wave. The diurnal maximal ratio between late and early T wave peak amplitude was found to correlate with a history of LQTS-related symptoms better than the baseline QTc interval in both LQT1 and LQT2 patients, and the diurnal distributions of maximal T2-to-T1 wave amplitude ratios were similar to those of cardiac events.
Diagnosis of the Long QT Syndrome
According to the most recent consensus statement, LQTS can be diagnosed in individuals testing positive for an unequivocally pathogenic mutation in one of the LQTS genes. In the absence of a pathogenic mutation, and in the absence of a secondary cause for QT prolongation, the diagnosis of LQTS requires the presence of one of the following: (1) QTc interval of at least 500 milliseconds (using the Bazett formula) on repeated 12-lead ECGs; (2) QTc interval between 480 and 499 milliseconds on repeated 12-lead ECGs in conjunction with unexplained syncope; or (3) Schwartz score of at least 3.5 points (see later).
In addition, LQTS can be suspected in individuals with QTc values exceeding 450 milliseconds for men and exceeding 460 milliseconds for women; the probability for LQTS increases if these subjects also have a history of syncope and familial SCD. On the other hand, LQTS is very unlikely among men with QTc less than 390 milliseconds and among women with QTc less than 400 milliseconds.
It is important to note that the LQTS is largely a clinical diagnosis, primarily based on the clinical presentation, personal and family history, and ECG findings. A detailed family history of syncope and SCD is essential, not only in first-degree relatives (mother, father, siblings, children), but also in more distant relatives. Also, the family history should also be investigated for other potential manifestations of malignant arrhythmias that might not have been classified as cardiac in origin, such as drowning, death while driving, epileptic seizures, and SIDS. Data on comorbidities in evaluated individuals or family members (such as congenital deafness) should also be acquired. Clinical features such as triggers of syncope and specific QT morphological attributes in patients in whom the clinical diagnosis has been made can suggest the affected gene in 70% to 90% of patients.
Importantly, a significant proportion (20% to 40%) of patients with genetically proven LQTS have normal or borderline QTc measurements at rest (“concealed” LQTS). It has been reported that these silent mutation carriers account for 36% of LQT1 patients, 19% of LQT2 patients, and 10% of LQT3 patients. Therefore the diagnosis of LQTS should not be excluded based solely on a QTc interval in the normal range (400 to 450 milliseconds), and additional testing is indicated whenever the clinical history requires exclusion of LQTS. In this setting, obtaining a resting ECG periodically and ambulatory ECG monitoring can sometimes uncover abnormal prolongation of the QTc interval, given the considerable day-to-day variability in QTc of patients with LQTS. In addition, reviewing the ECGs of all family members can be valuable because some family members can have obvious QT prolongation. The probability of LQTS in an index subject can be augmented if one of those family members has QTc prolongation.
Several provocative tests have been used to unmask LQTS patients with normal QTc on resting ECG. These include QT measurement upon abrupt standing, in the recovery phase of exercise testing, or during infusion of epinephrine. Also, genetic testing is recommended in patients with a strong clinical index of suspicion for LQTS. Although these diagnostic tools can all contribute to identifying patients with LQTS, a gold standard diagnostic tool is still lacking. Invasive EP testing is generally not useful in the diagnosis of LQTS.
Clinical Scoring Systems
When the diagnosis of LQTS is suspected but uncertain, a clinical scoring system (Schwartz score) has been developed to enhance the diagnostic reliability of clinical parameters and to estimate the probability of LQTS. The most recent (2011) update of the Schwartz score incorporates ECG features in combination with personal clinical history and family history. Scores were arbitrarily divided into three probability categories, providing a quantitative estimate of the risk for LQTS ( Table 31.5 ). A score of 3.5 or higher makes the diagnosis of LQTS very likely and should compel further investigation, including genetic testing when available. Among patients with suspected LQTS (based on QTc interval prolongation or clinical history), “high probability” of LQTS (score greater than or equal to 3.5) using the Schwartz criteria identified LQTS mutation carriers with a sensitivity of 89% and a specificity of 82%. Further testing is required in the group with “intermediate probability” of LQTS to confirm or exclude the diagnosis, including serial ECGs, Holter recordings, and provocative testing.
Points | |||
---|---|---|---|
Electrocardiographic Findings a | |||
A | QTc b | ≥480 msec | 3 |
460–479 msec | 2 | ||
450–459 (male) msec | 1 | ||
B | QTc b fourth minute of recovery from exercise stress test ≥480 msec | 1 | |
C | Torsade de pointes c | 2 | |
D | T wave alternans | 1 | |
E | Notched T wave in three leads | 1 | |
F | Low heart rate for age d | 0.5 | |
Clinical History | |||
A | Syncope c | With stress | 2 |
Without stress | 1 | ||
B | Congenital deafness | 0.5 | |
Family History | |||
A | Family members with definite LQTS e | 1 | |
B | Unexplained sudden cardiac death younger than age 30 among immediate family members e | 0.5 |
a In the absence of medications or disorders known to affect these electrocardiographic features.
b QTc calculated by the Bazett formula where QTc = QT/√RR.
d Resting heart rate below the second percentile for age.
e The same family member cannot be counted in A and B. (From Di Fusco SA, Palazzo S, Colivicchi F, Santini M. The influence of gender on heart rhythm disease. Pacing Clin Electrophysiol. 2014;37:650–657.)
Ambulatory Cardiac Monitoring
Holter monitoring is not sufficiently well standardized to serve in the primary assessment for ventricular repolarization analysis, and only rarely will show spontaneous arrhythmias in LQTS patients. However, this method can sometimes be used for the detection of extreme QT interval events that occur infrequently during the day. An important value of Holter recordings lies in showing T wave changes characteristic of LQTS, which can become evident especially during sleep or following post-extrasystolic pauses.
A recent study described a novel computer algorithm that measures QTc in a beat-to-beat manner during 24-hour Holter monitoring. The QTc measurements for a population or for an individual patient are displayed on a plot (“QTc clock” plot) to illustrate QT dynamics and characterize the extent and duration of QT prolongation. Using this technology, unique patterns of QT prolongation were observed in patients with LQT1 and LQT2. LQT1 patients were more likely to have diagnostic QTc prolongation during the daytime hours than during the night, likely correlating with the level of sympathetic activity ( eFig. 31.3 ). Conversely, LQT2 patients showed more QT prolongation during the night. Whether these observations can be used to distinguish between the different LQTS genotypes or to predict arrhythmogenic risk requires further investigation.

QT Interval Response to Abrupt Standing
The brief tachycardia associated with abrupt standing can potentially uncover patients with insufficient QT adaptation abilities and poor repolarization reserve, which characterize LQTS. Initially, an ECG is obtained in the supine position; then, the patient is asked to stand up briskly and a second ECG is obtained.
In one report, postural-induced QTc prolongation was more than 30 milliseconds in 68% of patients with “concealed” LQTS. Also, a QTc cutoff value of 490 milliseconds yielded a sensitivity of 89% and a specificity of 87% for the diagnosis of LQTS in patients with no excessive prolongation of the supine QT intervals (i.e., less than 480 milliseconds for females, less than 470 milliseconds for males). Postural QTc prolongation can be attenuated with beta-blockade.
Furthermore, T wave morphology changes exposed by quick standing are useful for diagnosing LQTS. The diagnosis of LQTS can be made with a higher degree of confidence when QT prolongation is accompanied by abnormal T wave morphology. The value of postural changes of T wave morphology for determining the specific LQTS genotype is modest, being most useful for LQT2, less useful for LQT1, and least useful for LQT3.
QT Interval Response to Exercise
Exercise testing can be useful to assess QT adaptation to heart rate, a measure of the integrity of the I Ks channels. Changes in QTc duration and prolonged QT hysteresis during exercise testing can be helpful in identifying patients with LQTS and even in predicting the genotype. Gradual supine bicycle testing can help minimize signal artifact from upper body motion observed during treadmill exercise.
In particular, the QTc at 4 minutes of recovery after maximal exercise discriminates between patients with LQTS and healthy subjects. In one study of patients with normal or borderline QTc intervals at baseline (i.e., less than 480 milliseconds for females, less than 470 milliseconds for males), a QTc greater than or equal to 480 milliseconds during the fourth minute of recovery identified LQTS patients with a sensitivity of 94% and a specificity of 90%. However, these diagnostic strategies have not been meticulously studied in an unselected population suspected of LQTS.
In addition, a gene-specific QTc interval behavior during exercise has been reported. Genetic mutations in LQT1 result in reduction of the amplitude of I Ks , one of the dominant K + currents responsible for repolarization especially at rapid heart rates. Attenuation of I Ks results in failure of the QT to adapt (i.e., failure to shorten) in response to increasing heart rate. A maladaptive, paradoxical prolongation of the QTc interval during the recovery phase (QTc greater than 470 milliseconds or a ΔQTc [QTc at 3 minutes of recovery minus the baseline supine QTc] greater than 30 milliseconds) was found to distinguish patients with manifest or concealed LQT1 from normal subjects and those with LQT2 and LQT3 genotypes.
In contrast, patients with LQT2 mutations have normal QT shortening or minimal QTc prolongation during exercise, but they characteristically demonstrate an exaggerated QT hysteresis compared with LQT1 patients and normal subjects. QT hysteresis is normally measured by comparing the QT intervals during exercise versus the recovery period at comparable heart rates (e.g., when the heart rate accelerates to approximately 100 beats/min during early exercise, and when the heart rate decelerates to approximately 100 beats/min during the recovery phase). In LQT2 patients, the QT fails to shorten at these intermediate heart rates in early exercise because of attenuated I Kr (a so-called I Kr zone). This is followed by recruitment of the unimpaired, sympathetically responsive I Ks , resulting in appropriate QT shortening at faster heart rates through to peak exercise, which persists into the recovery phase. This leads to increased QT hysteresis, that is, the QT interval is significantly longer during exercise than during recovery at comparable heart rates.
The LQT3 phenotype is characterized by a constant shortening of the QT interval with exercise because of stimulation of the intact I Ks channel and augmentation of a late inward Na + current. In fact, the QT interval in LQT3 patients shortens during exercise much more in LQT1 and LQT2 patients and even more than in healthy controls.
In addition, exercise treadmill testing can reveal the characteristic T wave morphology in patients with LQT1 and LQT2 syndromes.
Exercise-induced QTc prolongation and QT hysteresis can be attenuated with beta-blockade; therefore beta-blocker therapy should be discontinued before exercise testing.
Importantly, induction of arrhythmias during exercise is very rare in LQTS patients. Exercise-induced ventricular ectopy exceeding isolated PVCs is observed in less than 10% of patients. The presence of exercise-induced ventricular ectopy beyond single, isolated PVCs must prompt intense evaluation because it was found to have a positive predictive value exceeding 90% for the presence of significant cardiac pathology. However, CPVT, rather than LQTS, is the far more likely diagnosis.
Epinephrine QT Stress Test
Catecholamine provocation testing can help diagnose patients with concealed LQT1, with a positive predictive value approaching 75% and a negative predictive value of 96%. Furthermore, epinephrine provocation testing was found to be a powerful test to predict the genotype of LQT1, LQT2, and LQT3 syndromes.
Two major protocols have been developed for epinephrine infusion. Using the “escalating-dose infusion protocol,” epinephrine infusion is initiated at 0.025 µg/kg per minute and then increased sequentially every 10 minutes to 0.05, 0.1, and 0.2 µg/kg per minute. The 12-lead ECG is continuously recorded during sinus rhythm under baseline conditions and during epinephrine infusion. The QT interval is measured 5 minutes after each dose increase. Epinephrine infusion should be stopped for systolic blood pressure greater than 200 mm Hg, sustained or nonsustained VT, frequent PVCs (greater than 10 per minute), T wave alternans, or patient intolerance. A paradoxical QT interval response (prolongation of the absolute QT interval of greater than or equal to 30 milliseconds) during low-dose epinephrine infusion provides a presumptive clinical diagnosis of LQT1, with a positive predictive value of 75%. The diagnostic accuracy can be reduced in patients receiving beta-blockers.
Using the “bolus and infusion protocol,” an epinephrine bolus (0.1 µg/kg) is administered and immediately followed by continuous infusion (0.1 µg/kg per minute) for 5 minutes. The QT interval is measured 1 to 2 minutes after the start of epinephrine infusion when the R-R interval is the shortest (which represents the peak epinephrine effect) and 3 to 5 minutes after the start of epinephrine infusion (which represents the steady-state epinephrine effect). During the epinephrine test, patients with LQT1 manifest prolongation of the QTc at the peak of the epinephrine effect, which is maintained under steady-state conditions of epinephrine. In contrast, epinephrine prolongs the QTc more dramatically at the peak of epinephrine infusion in LQT2 patients, but the QTc returns to baseline levels under steady-state conditions. A much milder prolongation of QTc at the peak of epinephrine has been described in LQT3 patients and in healthy subjects, and it returns to the baseline levels under steady-state conditions. A subject is considered to have an LQT1 response if the QTc increase in the peak phase is greater than 35 milliseconds and is maintained throughout the steady-state phase ( Fig. 31.6 ). LQT2 response is likely if the peak QTc increase of greater than 80 milliseconds is not maintained in the steady-state phase. In one report, the sensitivity and specificity of the epinephrine test to differentiate LQT1 from LQT2 were 97% and 96%, those from LQT3 were 97% and 100%, and those from healthy subjects were 97% and 100%, respectively, when ΔQTc greater than 35 milliseconds at steady state was used. The sensitivity and specificity to differentiate LQT2 from LQT3 or healthy subjects were 100% and 100%, respectively, when ΔQTc greater than 80 milliseconds at peak was used.

The escalating-dose infusion protocol is generally better tolerated by the patient and carries a lower incidence of false-positive responses. On the other hand, the bolus and infusion protocol offers the ability to monitor the temporal course of the epinephrine response at peak dose (during the bolus) and during steady state (during the infusion), which is particularly important in individuals with LQT2 in whom transient prolongation of the uncorrected QT interval can occur, followed by subsequent shortening.
Genetic Testing
Although the diagnosis of LQTS can frequently be certain based on clinical diagnostic measures, genetic testing can still be of value; identification of the specific disease-causing mutation can potentially guide therapeutic choice, enhance risk stratification, and facilitate the identification of affected family members and implementation of lifestyle adjustment and presymptomatic treatment. Furthermore, genetic testing may be important in the identification of concealed LQTS, because a significant proportion (25% to 50%) of individuals with genetically proven LQTS can have a nondiagnostic QTc.
However, genetic testing remains expensive. Depending on the stringency of clinical phenotype assessment, the yield for positive genetic results in LQTS ranges from 50% to 78%, and is highest among tested individuals with the highest clinical probability (i.e., those with longer QTc intervals and more severe symptoms). The remaining probands with a strong clinical probability of LQTS will have a negative genetic test result, probably because of technical difficulties with genotyping, noncoding variants, or as yet unidentified disease-associated genes. Therefore a negative genetic test in a subject with clinical LQTS (i.e., genotype-negative/phenotype-positive LQTS) does not provide a basis to exclude the diagnosis. Nonetheless, a negative genetic test renders the diagnosis of LQTS very unlikely in those patients with low to moderate pretest probability based on clinical findings.
There is also the potential for false-positive results; genetic testing may identify novel mutations of unclear significance, which could represent normal variants, and require validation and further analysis (e.g., linkage within a family or in vitro studies).
Currently, comprehensive or targeted (LQT1, LQT2, and LQT3) genetic testing is recommended for symptomatic patients with a strong clinical index of suspicion for LQTS (Schwartz score greater than or equal to 3.5 points) and for asymptomatic patients with QT prolongation (QTc greater than or equal to 480 milliseconds [prepuberty] or greater than or equal to 500 milliseconds [adults]) in the absence of other clinical conditions that might prolong the QT interval. In addition, LQTS genetic testing may be considered for the asymptomatic subject with a QTc greater than or equal to 470 milliseconds on serial ECGs but with negative family history.
Differential Diagnosis: Acquired Long QT Syndrome
It is important to distinguish acquired factors that result in QT interval prolongation from the inherited form of LQTS. Acquired LQTS is far more prevalent than the congenital form. However, the occurrence of torsade de pointes is far less common than that of QT prolongation. Nonetheless, there is an increased risk for torsade de pointes whenever the QTc interval exceeds 500 milliseconds and whenever the QTc interval increases by more than 60 milliseconds from baseline, especially when the increase occurs rapidly.
In contrast to the most common types of congenital LQTS (LQT1 and LQT2), a short-long-short pattern of R-R cycles constitutes the typical pattern of initiation of torsades de pointes in acquired LQTS. The long pause exaggerates the QT interval prolongation, and the short-long-short sequence is thought to promote torsades de pointes by increasing transmural dispersion of repolarization. The short-long R-R interval is usually caused by a short-coupled PVC followed by a compensatory pause and then another PVC occurring during the T wave. However, because of the underlying QT interval prolongation, this R-on-T PVC does not have the short coupling interval that is characteristic of idiopathic VF. Torsades de pointes can also occur in association with bradycardia or frequent pauses (sometimes referred to as “pause-dependent LQTS”).
Etiology
Acquired causes of abnormal prolongation of the QT interval include myocardial ischemia, cardiomyopathies, electrolyte abnormalities (hypokalemia, hypomagnesemia, and hypocalcemia), autonomic influences, drugs, hypothyroidism, hypothermia, pheochromocytoma, intracranial bleeding, and bradycardia.
Hypokalemia causes prolongation of the action potential duration because of reductions in multiple K + currents including I Kr , I K1 , and I to . Low extracellular K + levels accelerate fast inactivation of the hERG channel and further decrease I Kr .
Acquired LQTS is also observed in the setting of extreme bradycardia, in particular, bradycardia complicating acquired AV block (spontaneous or iatrogenic). In fact, the original description of torsade de pointes, the hallmark arrhythmia of the LQTS, was entirely based on patients with LQTS complicating atrioventricular block. It is important to note that QT prolongation in patients with complete AV block is more pronounced than that in response to similar degrees of sinus bradycardia, and it is the magnitude of this QT prolongation in response to bradycardia, rather than the bradycardia per se, that determines the risk of torsade de pointes. It has been proposed that a change in QRS morphology, commonly observed in the setting of complete AV block but not during SND, leads to T wave changes associated with cardiac memory, which contributes to excessive QT prolongation. In one report, patients who developed a change in QRS morphology at the time of AV block had a sevenfold higher incidence of torsades de pointes and a change in the QRS axis seemed to be associated with an even greater incidence. These observations suggest that repolarization changes influenced by cardiac memory play a role in arrhythmia risk during AV block. Also, premature beats that lead to short-long-short cycles can foster the development of torsade de pointes.
By far, the most common environmental stressor resulting in acquired LQTS is drug therapy, including antiarrhythmic drugs, some antihistamines, antipsychotics, and antibiotics ( www.qtdrugs.org ). Noncardiovascular drugs that can potentially precipitate QT interval prolongation and arrhythmias comprise approximately 2% to 3% of total prescribed medications. Indeed, the risk of acquired LQTS is the most common cause of withdrawal or restriction of drugs that have already been marketed. For specific drugs, the incidence of torsade de pointes is difficult to quantify, and ranges from extremely low for many of the macrolide antibiotics, to 0.5% to 1% for drugs such as sotalol and azimilide, to 1.5% to 9% for quinidine.
The vast majority of drugs associated with the acquired form of LQTS are known to interact with the hERG channel (which mediates I Kr ), likely because of unique structural properties rendering this channel unusually susceptible to blockade by a wide range of different drugs. Compared with other cardiac K + channels, the hERG channel has a large, funnel-like vestibule that allows many small-sized molecules to enter and block the channel ( see Chapter 2 ). The more spacious inner cavity is due to the lack of the Pro-X-Pro sequence motif in the S6 segment (which is present in most other voltage-gated K + channels and is believed to induce a sharp bend in the inner S6 helices of voltage-gated K + channels, reducing the inner vestibule), presumably facilitates access of drugs to the pore region from the intracellular side of the channel to block the channel current. In addition, the hERG channel contains two aromatic sites inside its pore (not present in most other K + channels), which provide high-affinity binding sites for aromatic moieties of a wide range of structurally diverse compounds. Interaction of these compounds with the channel’s pore causes functional alteration of its biophysical properties, occlusion of the permeation pathway, or both.
Other mechanisms underlying drug-induced LQTS have been recently described, including disruption of hERG channel protein trafficking, with consequent reduction of surface membrane expression of otherwise functional channels (e.g., pentamidine), or folding and assembly of channel subunits. The accessory β-subunit (MiRP1, KCNE2 ) of the hERG channel also determines the drug sensitivity.
Risk Factors of Drug-Induced Long QT Syndrome
Several factors can potentially increase the susceptibility to drug-induced LQTS, including female gender (70% of patients with drug-induced torsades de pointes are women), advanced age, hypokalemia, hypomagnesemia, hypocalcemia, bradycardia, congestive heart failure, LV hypertrophy, recent conversion from AF, and the presence of QT interval prolongation on baseline ECG. Other risk factors include high drug doses (with the exception of quinidine), rapid IV infusion, and concurrent use of other drugs that prolong the QT interval or promote other QT-prolonging factors (such as bradycardia or electrolyte abnormalities). Also, conditions leading to accumulation of QT-prolonging drugs in plasma, such as from drug-drug interactions, are in general risk factors for torsades de pointes. These include the presence of renal or hepatic failure and the concomitant use of drugs that slow drug metabolism or impair drug excretion. In addition, genetically determined variability in pharmacodynamics (e.g., polymorphisms and mutations of the cytochrome system) can be responsible for significant variations in drug response. Most patients with drug-induced torsades de pointes have one or more risk factors.
Hospitalized patients appear to be at greater risk of drug-induced QT interval prolongation and torsades de pointes than outpatients, likely due to a greater preponderance of risk factors, including structural heart disease, advanced age, electrolyte abnormalities, bradycardia, or renal or hepatic disease. A risk score was developed for predicting the risk of developing acquired LQTS in hospitalized patients in cardiac care units ( Table 31.6 ). The risk score effectively distinguished hospitalized patients at moderate or high risk for QTc interval prolongation from those at low risk. The occurrence of drug-induced torsades de pointes is an extremely rare event in patients without any risk factors. Over 90% of patients who develop torsades de pointes have at least one, and 71% of patients have at least two risk factors.
Risk Factor Points | |
---|---|
Age ≥68 years | 1 |
Female sex | 1 |
Loop diuretic | 1 |
Serum potassium ≤3.5 mEq/L | 2 |
Admission QTc ≥450 msec | 2 |
Acute myocardial infarction | 2 |
≥2 QTc-prolonging drugs | 3 |
Sepsis | 3 |
Heart failure | 3 |
One QTc-prolonging drug | 3 |
Maximum Risk Score | 21 |
Genetics
Evidence suggests that drug-induced LQTS can represent a “forme fruste” of congenital LQTS in a significant proportion of patients, whereby drug challenge merely exposes the presence of the congenital form of the syndrome. This is likely related to a redundancy in repolarizing currents; normal repolarization is accomplished by multiple ion channels, providing a safety reserve for repolarization (the concept of “repolarization reserve”), which is genetically determined and compensates for any factor (e.g., drugs or genetic mutation) that might either decrease repolarizing or increase depolarizing currents during the action potential. As a result, a mutation or polymorphism in one of the LQTS genes can be clinically inapparent until another insult to repolarization, such as a drug, hypokalemia, or hypomagnesemia, is superimposed.
Mutations of ion channel genes responsible for LQTS have been implicated as a risk factor. In fact, previously unrecognized congenital LQTS, of any subtype, can be identified in as many as 36% to 40% of drug-induced LQTS patients. In addition, polymorphisms (i.e., common genetic variations present in greater than 1% of the population) in cardiac ion channels can potentially increase the risk for the development of drug-induced torsades de pointes. In a recent study, drug-induced LQTS patients had a greater burden of amino acid coding variants (missense, nonsynonymous, or frameshift), than the control subjects, suggesting that multiple rare variants, notably across congenital LQTS genes, predispose to drug-induced LQTS.
In a large cohort of acquired LQTS subjects, the QTc interval remained prolonged (although to a milder degree than that observed in congenital LQTS carriers) in a significant proportion of patients even after the QT-prolonging factors are removed (withdrawal of culprit drugs or correction of serum electrolytes), suggesting that acquired LQTS could represent at least in part a latent genetic predisposition. Further, 28% of acquired LQTS subjects were found to harbor mutations in one of the major LQTS-related genes (most commonly in the KCNH2 gene). Even among the “true” acquired LQTS, i.e., those with normal QT interval outside the triggering episode, 23% had congenital LQTS-causing mutations. Predictors for carrying a pathogenic mutation in acquired LQTS included (1) age less than 40 years, (2) baseline QTc interval exceeding 440 milliseconds, and (3) history of symptoms at the time of acquired LQTS diagnosis ( Table 31.7 ). Of the acquired LQTS patients carrying a mutation, 88% had two or more of those predictors, while only 3% of patients with one or none of those risk factors had a mutation. Therefore some investigators have proposed genetic screening in acquired LQTS subjects with two or more of the risk factors to enable “cascade screening” in their family members and thereby prevent avoidable risks of life-threatening arrhythmias.
OR (95% CI) | P Value | |
---|---|---|
Age, <40 vs. ≥40 years | 2.5 (1.2–5.3) | 0.020 |
QTc, >440 vs. ≤440 msec | 5.2 (2.2–12.2) | <0.001 |
Clinical status, symptomatic vs. asymptomatic | 10.6 (1.3–83.5) | 0.025 |
Management
First-line treatment approaches to torsades de pointes include removal of the offending causes, the use of IV magnesium, maintenance of high-normal serum K + level, and avoidance of QT-prolonging agents. In some refractory cases, isoproterenol infusion or temporary transvenous overdrive pacing may be required to increase heart rate, which can shorten the QT interval and suppress torsades de pointes.
Magnesium suppresses torsades de pointes without shortening the QT interval, presumably by suppressing the EADs that trigger torsades de pointes. EAD suppression by magnesium has been attributed to its Ca 2+ channel–blocking effects but may also be mediated by a reduction of the late component of the sodium current (I NaL ). The value of acute K + repletion is less well documented than that of IV magnesium for the acute treatment of drug-induced torsades de pointes. Normal levels of both potassium and magnesium should be maintained aggressively in hospitalized patients at risk.
Recent studies suggested that mexiletine (a strong I NaL blocker) may be an effective treatment for acute termination of torsades de pointes in several acquired causes of QT interval prolongation. Although torsades de pointes often responds to a lidocaine bolus, arrhythmias tend to recur despite continuous infusions. In contrast, ranolazine (an I NaL blocker), flecainide (a strong I Na blocker), and verapamil (a strong I CaL blocker) also block I Kr , thus limiting their use in patients with drug-induced LQTS. Of note, a recent report demonstrated potential benefit of oral progesterone for the prevention of drug-induced QT interval prolongation.
Risk Stratification
The clinical course in LQTS patients is not uniform and is influenced by many factors, including age, gender, genotype, environmental factors, therapy, and possibly other modifier genes.
Clinical Markers of Risk
Syncope.
Nonfatal events (syncope and aborted cardiac arrest) in LQTS patients remain the strongest predictor of subsequent LQTS-related fatal events, and the overall risk of subsequent SCD in an LQTS patient who has experienced a previous episode of syncope is approximately 5% per year.
The risk of subsequent syncopal episodes can be reduced with beta-blocker therapy; however, patients experiencing syncope while receiving beta-blockers are at high risk of subsequent cardiac events, a risk similar to that observed in patients who are not treated with beta-blockers.
Both the timing and frequency of syncopal events are related to the subsequent risk of cardiac events. Patients with recent (within the past 2 years) syncope and a higher number of syncopal events during this period carry a higher risk of subsequent cardiac events.
However, it is important to distinguish between suspected arrhythmogenic and nonarrhythmogenic causes of syncope, especially given the fact that neurocardiogenic syncope is not uncommon in this patient population and its occurrence does not impact the prognosis negatively. A detailed clinical history in LQTS patients presenting with syncope is imperative, since clinical features (in particular, the occurrence of prodromes and the presence of specific triggers) can allow distinction between suspected arrhythmogenic and nonarrhythmogenic etiologies in a large proportion of cases.
Family history.
The incomplete penetrance and variable expressivity in LQTS preclude predicting severity of symptoms in relatives of a symptomatic LQTS patient. In fact, SCD of a sibling (at any age) does not seem to contribute to increased personal risk of LQTS-related life-threatening cardiac events. Instead, the risk of adverse events in relatives appears to be determined more by the individual’s own risk factors (QTc duration, personal history of syncope, and gender).
Gender.
The effect of gender on outcome is age dependent, with boys exhibiting a significant (71% to 85%) increase in risk for syncope, aborted cardiac arrest, or SCD as compared with girls during childhood and early adolescence. However, a gender risk reversal occurs after age 14 years, in which girls exhibit an 87% increase in the risk of cardiac events compared with boys among probands and a 3.3-fold increase in the risk among affected family members. When only life-threatening cardiac events (aborted cardiac arrest or SCD) are considered, the onset of gender risk reversal occurs at a later age. The cumulative probability of a first life-threatening cardiac event from age 1 to 12 years is 5% in boys compared with only 1% among girls, whereas in the age range of 12 to 20 years, there is no significant gender difference in risk. Risk reversal for the endpoint of aborted cardiac arrest or SCD occurs after the age of 20 years, and women maintain higher risk than men throughout adulthood.
Importantly, the effect of gender on outcome appears to be genotype dependent. Among those younger than 15 years, male LQT1 patients exhibit the highest risk of cardiac events; female LQT1 patients had intermediate risk; and both male and female LQT2 patients had the lowest risk. No significant gender-related difference in risk was shown among LQT2 and LQT3 children. In contrast, in the 15- through 40-year-old age group, the risk is highest among LQT2 women, intermediate among LQT1 women, and lowest among LQT1 and LQT2 men.
Age.
Risk factors in LQTS are time dependent and age specific ( Table 31.8 ). LQTS patients who experience an aborted cardiac arrest during the first year of life are at a very high risk for subsequent life-threatening cardiac events during the first decade of life. The risk factors for a cardiac event in LQTS infants also include a QTc of at least 500 milliseconds, a heart rate not exceeding 100 beats/min, and female gender.
Age Group | Risk Factor | Hazard Ratio ( P Value) | Beta-Blocker Efficacy, % Reduction ( P Value) |
---|---|---|---|
Childhood (1–12 years) | Male gender | 3.96 (<0.001) | 73% (0.002) |
QTc >500 msec | 2.12 (0.02) | ||
Prior syncope | |||
Recent (>2 years) | 14.34 (<0.001) | ||
Remote (≥2 years) | 6.45 (<0.001) | ||
Adolescence (10–20 years) | QTc >530 msec | 2.3 (<0.001) | 64% (0.01) |
Syncope | |||
≥2 syncopal events in past 2 years | 18.1 (<0.001) | ||
1 syncopal event in past 2 years | 11.7 (<0.001) | ||
≥2 syncopal events in past 2–10 years | 5.8 (<0.001) | ||
1 syncopal events in past 2–10 years | 2.7 (<0.001) | ||
Adulthood (18–40 years) | Female gender | 2.68 (<0.05) | 60% (<0.01) |
QTc duration | |||
QTc ≥500 msec | 6.35 (<0.01) | ||
QTc = 500–549 msec | 3.34 (<0.01) | ||
Prior syncope | 5.10 (<0.01) | ||
Adulthood (41–60 years) b | Recent syncope (<2 years) | 9.92 (<0.001) | 42% (0.40) c |
QTc > 530 msec | 1.68 (0.06) | ||
LQT3 genotype | 4.76 (0.02) |
a Findings are from separate multivariable Cox models in each age group for the endpoint of aborted cardiac arrest or sudden cardiac death.
b Because long QT syndrome (LQTS)–related events are more difficult to delineate in the older age group, the endpoint in the 41- to 60-year-old age group comprised aborted cardiac arrest or death from any cause.
c Lack of a statistically significant beta-blocker effect in this age group may relate to the broad endpoint of death from any cause.
In LQTS children, risk factors for life-threatening cardiac events include male gender, a history of syncope at any time during childhood, and a QTc duration greater than 500 milliseconds.
Among adolescent patients with suspected LQTS, recent episodes of syncope (in particular within the past 2 years) and QTc greater than 530 milliseconds predict increased risk of LQTS-related cardiac events. Although LQT1 genotype predicts higher risk in patients not more than 14 years of age (especially boys), the risk is higher for LQT2 in patients 15 years of age or older (especially women).
In adult patients, predictors of worse outcome include a QTc greater than 500 milliseconds, female gender, and history of syncope before age 18 years. In addition, patients with LQT2 mutations appear to be at a greater risk for a cardiac event than patients with LQT1 or LQT3 genotypes.
Beyond 40 years of age, recent syncope (within the past 2 years) appears to be the predominant risk factor in affected subjects, and those with a positive mutation had a significantly higher mortality, particularly those with an LQT3 mutation. Furthermore, women with a QTc greater than 470 milliseconds are at a higher risk of LQTS-related cardiac events, whereas in men, event rates are similar in the various QTc categories. After the age of 60 years, the risk of death due to LQTS competes with other disease entities that may lead to death.
Electrocardiographic Markers of Risk
QT interval prolongation.
The QTc interval is the best prognostic ECG parameter in LQTS families. A QTc interval of at least 470 milliseconds is a predictor for increased risk for symptoms, whereas a QTc of at least 500 milliseconds predicts an increased risk of life-threatening cardiac events. Although there is no threshold of QTc prolongation at which torsades de pointes is certain to occur, a gradual increase in risk for torsades de pointes is observed as the QTc interval increases. Each 10-millisecond increase in QTc contributes approximately a 5% to 7% additional increase in risk for torsades de pointes. Therefore a patient with a QTc of 540 milliseconds has a 63% to 97% higher risk of developing torsades de pointes than a patient with a QTc of 440 milliseconds.
Importantly, considerable time-dependent variability and instability of ventricular repolarization exist in LQTS. Thus risk stratification should take into account the risk associated with variability in the duration of the QTc interval in the individual LQTS patient during follow-up. In fact, it has been shown that the maximum QTc duration measured at any time before age 10 was the most powerful predictor of cardiac events during adolescence, regardless of baseline, mean, or most recent QTc values.
Furthermore, a considerable variability in the duration of the QTc interval exists among LQTS patients with identical mutations. Evidence suggests that mutation-specific QTc variability (defined as QTc standard deviation [QTcSD] among all carriers of a specific mutation) provide incremental prognostic information for risk stratification that is independent of those related to the individual’s own QTc. A greater degree of repolarization instability associated with specific LQTS mutations (manifesting as a high mutation-specific QTcSD) was identified as an independent risk predictor even in high-risk patients with long QTc durations. It has been reported that every 20-millisecond increment in mutation-specific QTcSD resulted in a 33% increased risk for cardiac events after adjustment for the patient-specific QTc, and patients who had mutations with QTcSD of 45 milliseconds or more experienced a significant 48% increase in the risk of cardiac events compared with patients who had mutations with QTcSD less than 45 milliseconds. These findings emphasize the importance of checking mutation-specific effects in various genetic backgrounds to identify mutations that are more susceptible to intrinsic or extrinsic modifying factors. It is important to note that the prognostic implications of QTcSD appear to be genotype specific; the risk associated with a higher QTcSD was pronounced among patients with LQT1, whereas in patients with LQT2, no significant association of QTcSD with cardiac events was found.
T-U wave morphology.
The ratio of the amplitude of the U wave to that of the T wave has been suggested as the clinical counterpart of EADs; a progressive increase in the ratio of the U wave to the T wave preceded the onset of torsades de pointes in an experimental model of LQTS. In addition, the increment in U wave amplitude after a PVC has been suggested as a marker for arrhythmia risk in “pause-dependent” LQTS.
In patients with bifid T waves, the diurnal maximal ratio between late and early T wave peak amplitude was found to correlate with a history of LQTS-related symptoms better than the baseline QTc interval in both LQT1 and LQT2 patients, and can potentially be used to assess the risk of symptoms in asymptomatic patients with known type 1 or 2 LQTS genotype. In LQT1 patients, a ratio of at least three suggests a high probability of being symptomatic whereas a ratio not exceeding two suggests a low probability. Among LQT2 patients, ratios suggestive of being symptomatic or asymptomatic are, respectively, at least 2.4 and not more than 1.5.
Of note, the presence of macroscopic T wave alternans (bidirectional beat-to-beat changes in T wave polarity) indicates electrical instability and high acute risk of ventricular arrhythmias.
Genetic Markers of Risk
The genotype is an important predictor of LQTS-related cardiac events. The risk of cardiac events has been shown to be significantly higher in LQT1 and LQT2 when compared with LQT3, with events occurring at a younger age. The cumulative mortality, however, appears to be similar regardless of the genotype, since patients with LQT3 develop arrhythmias less often, but these are more likely to be lethal when they ultimately occur.
LQT1 patients exhibit a 49% increase in the risk of cardiac events as compared with LQT2 patients. However, in the 15- through 40-year-old age group, the risk of a first cardiac event is significantly higher among LQT2 patients (67% increase in the risk as compared with LQT1 patients). Among LQTS patients receiving beta-blocker therapy, the LQT2 and LQT3 genotypes are associated with increased risk of arrhythmic events as compared with LQT1.
In addition to identifying the LQT genotype, knowing the specific mutation and its biophysical function can help improve risk stratification. For LQT1, patients with mutations in the transmembrane domain of the KCNQ1 channel had more frequent cardiac events (syncope, aborted cardiac arrest, or SCD) and a greater risk of the first cardiac event occurring at a younger age than did patients with C-terminal mutations. For LQT2, pore mutations have a more severe clinical course and a higher frequency of arrhythmic events occurring at a younger age when compared with nonpore mutations. In particular, missense mutations in the transmembrane pore (S5-loop-S6) region appear to be associated with the highest risk of clinical arrhythmia.
Preliminary data for LQT3 patients also suggest that the location and biophysical function of the mutation can play a role in determining the severity of the clinical phenotype. Mutations with a dominant-negative effect on ion channel function (greater than 50% reduction in function) have a more severe phenotype compared with mutations exhibiting haploinsufficiency (less than or equal to 50% reduction in function).
Jervell and Lange-Nielsen syndrome and the Timothy syndromes (LQT8) have a highly malignant course with poor prognosis, and are less likely to respond to beta-blocker therapy alone. In contrast, the Andersen-Tawil syndrome has a generally more benign clinical course in terms of arrhythmic death.
The presence of multiple mutations (the so-called “double hits,” observed in 8% to 11% of patients with LQTS) is associated with a significantly higher risk for life-threatening cardiac events as compared with patients with a single mutation. Also, among patients with multiple mutations, a double hit in a single gene (compound heterozygosity) was associated with a greater risk for life-threatening cardiac events than a double hit in different genes (digenic heterozygosity).
Principles of Management
The main therapeutic modalities for the prevention of life-threatening cardiac events include beta-blockers, left (sometimes bilateral) cervicothoracic sympathetic denervation, and implantable cardioverter-defibrillator (ICD) implantation. In nongenotyped patients, beta-blockers comprise the mainstay therapy, whereas cervicothoracic sympathetic denervation and ICD implantation are therapeutic options in high-risk LQTS patients who experience recurrent cardiac events despite beta-blocker therapy ( Table 31.9 ). Renal sympathetic denervation may play a future role.
Class I |
|
| |
| |
| |
| |
| |
| |
| |
| |
| |
| |
Class IIa |
|
| |
| |
| |
Class III |
|
Pharmacological Therapy
Treatment with beta-blockers is associated with a significant (53% to 64%) reduction in risk of LQTS-related life-threatening events (syncope, cardiac arrest, and SCD), regardless of age (see Table 31.8 ). The benefit appears to be most substantial in patients at highest risk for cardiac events. On the other hand, very low-risk patients (no history of syncope, QTc less than 500 milliseconds, girls younger than 14 years, LQT2 men of any age, and LQT1 women older than 14 years) have such a small frequency of events that beta-blockers may not provide a substantial benefit.
Genetic data can be used to guide the therapeutic management plan. Given the critical role of catecholamines in precipitating arrhythmias in LQT1, beta-blocker therapy is particularly effective for this group of patients; approximately 90% of LQT1 patients treated with beta-blockers remained free from syncope and cardiac arrest after a mean follow-up time of 5.4 years and showed a total mortality rate of 1%. Although beta-blockers were generally considered to have lower efficacy in LQT2 patients as compared with LQT1 patients, recent data argue for a similar magnitude of risk reduction in LQT2 patients (beta-blocker therapy decreased cardiac events from 58% to 23% after an average follow-up of 4.9 years on therapy). The higher residual event rate in LQT2 patients while receiving beta-blocker therapy is likely due to a higher overall event rate in patients with this genotype, rather than to an attenuated efficacy of medical therapy in this high-risk population. Of note, a trigger-specific response to beta-blocker therapy exists within the LQT2 population; beta-blockers appear to be more protective against exercise-triggered cardiac events than arousal- or non–exercise-related events (see Fig. 31.3 ).
On the other hand, the value of beta-blocker therapy in LQT3 patients is debated. These patients continue to experience high rates of cardiac events despite beta-blocker therapy. Among LQT3 patients receiving beta-blocker therapy, the adjusted risk for a cardiac event is fourfold higher than among LQT1 patients. However, when excluding LQT3 patients with the most severe phenotype (i.e., patients who had suffered a cardiac event during infancy and those with QTc greater than 600 milliseconds), beta-blocker therapy appears to be beneficial in LQT3. It is important to note, however, that prolongation of the QT intervals is aggravated at slow heart rates; thus a reduction in heart rate with beta-blockers can potentially pose a therapeutic problem in these patients.
Currently, beta-blockers are considered the mainstay therapy for the prevention of life-threatening cardiac events and, given the approximately 12% risk of SCD as the first manifestation of LQTS, they should be considered the first-line measure in all nongenotyped patients and in patients with LQT1 or LQT2 genotypes, regardless of their symptomatic or risk status ( Fig. 31.7 ). Possible exceptions could include very low-risk LQTS patients (based on clinical and ECG parameters) in whom beta-blocker therapy may be considered on an individual basis. That said, it is important to understand that all these recommendations are based on observational studies and not randomized clinical trials.

Propranolol and nadolol are the beta-blockers most widely used. Limited data exist regarding the effectiveness of other beta-blockers in preventing cardiac events in patients with LQTS, although some evidence suggests the superiority of nadolol and propranolol as compared to metoprolol in LQT1 and LQT2 patients. Long-acting medications are preferred to improve compliance and avoid of wide fluctuations in blood levels. The nonselective beta-blocker nadolol (which has strong negative chronotropic effect and long half-life [20 to 24 hours]) is the most preferred first-choice therapy in patients with LQTS. Propranolol is probably the preferred beta-blocker in LQT3 patients, given its direct late Na + current blocking properties.
The protective effect of beta-blockers is related to their adrenergic blockade, which diminishes the risk of cardiac arrhythmias; hence, the goal of beta-blocker therapy is to blunt the maximal heart rate during exercise, and the adequacy of beta-blockade should be assessed by exercise testing or ambulatory monitoring. It is recommended to start at a low dose and slowly up-titrate the dose (over several weeks, especially in asymptomatic patients) to help improve patient tolerance and compliance, which can potentially lead to otherwise unnecessary ICD implantation. Of note, beta-blockers do not substantially shorten the QT interval.
Despite the beneficial effects of beta-blockers, a high rate of residual cardiac events has been reported in patients receiving beta-blocker therapy, occurring in 10%, 23%, and 32% of LQT1, LQT2, and LQT3 patients, respectively, after a mean follow-up time of 5.4 years. Therefore patients who remain symptomatic despite treatment with beta-blockers should be considered for other therapeutic modalities. Interestingly, it has been reported that noncompliance is an important cause of events occurring during beta-blocker treatment in LQT1 patients.
Implantable Cardioverter-Defibrillator
ICD therapy is highly effective to prevent SCD in high-risk LQTS patients (mortality of 1.3% in high-risk ICD patients compared with 16% in non-ICD patients during a mean follow-up time of 8 years). Therefore ICD implantation should be considered for secondary prevention in patients with prior cardiac arrest and for primary prevention in those who experience unexplained syncope or ventricular tachyarrhythmias while receiving beta-blocker therapy ( Fig. 31.8 ).

Although prophylactic ICD therapy used to be considered for LQTS patients with risk factors for SCD (see Table 31.8 ) regardless of medical therapy, recent data suggest that high-risk LQT1 and LQT2 patients should be considered for prophylactic ICD implantation only if they develop recurrent cardiac events (e.g., syncope or torsades de pointes) despite beta-blocker therapy or when compliance with or intolerance to medical therapy is a concern. Using this approach, most patients with LQTS do not need an ICD. Clear understanding by the patient and family of the relative merits of each strategy is essential. Although beta-blocker therapy significantly reduces the risk of SCD in this population, it is not completely protective, and residual cardiac events still occur. On the other hand, data suggest that syncopal episodes almost always precede cardiac arrest in patients receiving beta-blockers, allowing for institution of other therapeutic modalities, such as ICD implantation. In addition, ICD therapy is not without complications; infection, lead malfunction, inappropriate shocks, psychological sequelae, as well as the need for periodic device or lead revisions, have to be taken into consideration. This is especially important in children and young adults in whom the initial decision to implant an ICD carries decades-long implications, such as multiple procedures for generator replacements and lead extractions, increasing the lifetime risks of morbidity and mortality.
Because ICD therapy does not prevent the occurrence of arrhythmias, concurrent treatment with beta-blockers is recommended for symptomatic and high-risk patients. Furthermore, careful programming of the ICD arrhythmia detection settings is recommended to reduce the risk of inappropriate shocks. This usually involves programming a single VF zone of more than 220 beats/min with or without a monitoring zone of more than 180 beats/min.
The European registry of 233 LQTS patients with ICDs showed that 28% received appropriate ICD therapies, and that future appropriate ICD therapies could be predicted by four variables: (1) age younger than 20 years at implantation; (2) QTc greater than 500 milliseconds; (3) prior cardiac arrest; and (4) cardiac events despite therapy. The M-FACT scoring system ( Table 31.10 ), based on those clinical variables, was developed to identify in advance those patients with the highest and lowest probability of receiving appropriate shocks, which might represent the justification for the ICD implantation. Within 7 years, appropriate ICD therapies did not occur in any patients who had none of these factors and did occur in 70% of patients with all four clinical factors. Patients with an M-FACT score of 0 are unlikely to benefit from ICD implantation.
Points | |
---|---|
Event-free on therapy for >10 years | −1 |
QTc | |
501–550 msec | 1 |
>550 msec | 2 |
Age at implantation ≤20 years | 1 |
Prior aborted cardiac arrest | 1 |
Events on therapy | 1 |
a Acronym derived from M (minus 1 point for being free of cardiac events while on therapy for >10 years), F (500- and 550-msec QTc), A (age ≤20 years at implantation), C (cardiac arrest), and T (events on therapy).
In addition, prophylactic ICD therapy should be considered in very-high-risk patients, including symptomatic patients with two or more gene mutations, including those with the Jervell and Lange-Nielsen syndrome variant with congenital deafness.
Left Cervicothoracic Sympathectomy
Left cervicothoracic sympathectomy, which involves resection of the lower half of the left stellate ganglion and the first two to four thoracic ganglia (T1–T4), is another antiadrenergic therapeutic option for patients with LQTS. Care must be taken to not damage the top half of the ganglia to avoid Horner syndrome.
Currently, left cervicothoracic sympathectomy is recommended for the management of high-risk LQTS patients in whom drug therapy may not be sufficiently protective when ICD therapy is refused or not feasible (e.g., small infants) and when beta-blockers are not effective, not tolerated, or contraindicated.
Although cardiac sympathetic denervation provides a significant long-term reduction in the frequency of aborted cardiac arrest and syncope (more effective in patients with LQT1 than in those with other types of LQTS), it is not completely protective against SCD. Studies of LQTS patients who have undergone left cervicothoracic sympathectomy demonstrated a residual mortality rate of 5% at 5 years. Furthermore, about 50% of high-risk LQTS patients have experienced one or more cardiac events, and patients with extremely malignant LQTS might not be responsive to cardiac sympathetic denervation. Hence, left cervicothoracic sympathectomy must not be viewed as curative or as an ICD alternative for high-risk patients.
Importantly, postoperative morbidity is common in patients undergoing left cervicothoracic sympathectomy. A large proportion of patients experience left-sided dryness, unilateral facial flush with exercise, contralateral hyperhidrosis, differential hand temperatures, transient and permanent ptosis, and left arm paresthesia. Nonetheless, postoperative satisfaction is generally high and patients feel safer following the procedure, despite the side effects.
Permanent Pacemaker
Cardiac pacing, in conjunction with beta-blocker therapy, can potentially reduce the risk of bradycardia-dependent QT prolongation, decrease heart-rate irregularities (eliminating short-long-short sequences), and reduce repolarization heterogeneity. Permanent pacemakers can be of value, especially in patients who continue to be symptomatic despite beta-blocker therapy or those in whom bradycardia or AV block limits the use of such therapy. In particular, patients with documented pause- or bradycardia-induced torsades de pointes and those with the LQT3 genotype may derive significant benefit from cardiac pacing.
Nevertheless, the high mortality in patients with recurrent symptoms (syncope or torsades de pointes) while receiving beta-blocker therapy is not adequately attenuated by the addition of cardiac pacing. Therefore if cardiac pacing is being considered, the use of an ICD is more logical because it provides protection from SCD as well as the benefit of cardiac pacing. However, when ICD implantation is associated with an extremely high rate of adverse events (such as in small infants), an atrial pacemaker in combination with beta-blocker therapy can potentially serve as a bridge to ICD placement.
When cardiac pacing is employed, atrial pacing is preferred, at a rate that shortens the QTc to less than 440 milliseconds. It is recommended to minimize ventricular pacing as possible because it can potentially increase the heterogeneity of ventricular repolarization. However, in patients with AV block, ventricular pacing is important to maintain AV synchrony and elimination of ventricular pauses and long-short cycles.
Catheter Ablation
In LQTS patients, frequent episodes of torsades de pointes are occasionally triggered by focal, monomorphic PVCs. In this setting, catheter ablation of the focus of the PVCs can be valuable in reducing the burden of arrhythmias and the frequency of ICD therapies.
Lifestyle Modifications
Education and lifestyle changes for the prevention of arrhythmias are critical in patients with LQTS. Patients should avoid drugs that prolong the QT interval ( www.qtdrugs.org ) or reduce their serum potassium or magnesium levels, and they should consult with their physician before taking any medications or over-the-counter supplements. Furthermore, patients need to be educated on conditions that can lead to potentially dangerous electrolyte abnormalities (e.g., dehydration, diarrhea, vomiting, imbalanced diets for weight loss).
In addition, the importance of compliance with medical therapy should be emphasized. Beta-blocker noncompliance and use of QT-prolonging drugs account for the vast majority of life-threatening events in LQT1 patients. Preventive measures in LQTS patients in general and LQT2 patients in particular include avoidance of unexpected auditory stimuli (such as alarm clocks, doorbells, and telephones), especially during rest or sleep. Families with LQTS may also consider basic life support training and operation of an automated external defibrillator.
Participation in Sports
Physical activity and stress-related emotions frequently trigger cardiac events in patients with LQTS, especially patients with LQT1 or LQT2. Therefore all competitive sports (except those in the class IA category, such as billiards, bowling, cricket, and golf) should be restricted in symptomatic LQTS patients (regardless of the QTc duration or underlying genotype) as well as asymptomatic patients with baseline QT prolongation (QTc greater than or equal to 470 milliseconds in men, greater than or equal to 480 milliseconds in women). Swimming is particularly hazardous in LQT1 patients and should therefore be limited or performed under appropriate supervision, even in subjects with genotype-positive/phenotype-negative LQT1.
Because many first cardiac events occur before the age of 15 years in male patients, particularly those with the LQT1 genotype, whereas female patients may experience first cardiac events after the age of 20 years, LQT1 male patients require stricter exercise restriction before the age of 15.
Recent data suggests that sports participation is safer than previously recognized, and the restriction limiting participation to class IA activities may be liberalized for the asymptomatic patient with genetically proven LQT3 genotype and those with genotype-positive/phenotype-negative LQTS (especially patients genotyped as non-LQT1 and no family history of multiple SCDs), assuming that appropriate disease-specific treatments are in place. In addition, automated external defibrillators and personnel trained in basic life support should be readily available. Furthermore, precautionary measures should be undertaken to avoid exercise-related dehydration, heat exhaustion, and electrolyte abnormalities.
Sports participation may also be carefully considered in LQTS patients with QT prolongation and those rendered asymptomatic after institution of treatment. These decisions, however, should be deliberated carefully by an LQTS expert to ensure that the athletes and their family members have been well informed, well risk stratified, and well treated.
Other considerations include the acquisition of a personal automatic external defibrillator as part of the athlete’s personal sports safety gear, and establishment of an emergency action plan with the appropriate training facility and team officials.
Gene-Specific Therapy
The standard therapeutic options for LQTS (including beta-blockers, cardiac sympathetic denervation, ICD) rely on genotype to only a minor degree, yet are quite effective. Nevertheless, beta-blockers and left cervicothoracic sympathectomy have some degree of genotype specificity, being quite effective in LQT1 and LQT2 and less effective in LQT3. Similarly, lifestyle modification and exercise restriction are most helpful in LQT1 and LQT2. For practical purposes, however, this apparent genotype specificity influences therapy decisions in only a very small number of patients.
Several gene-specific LQTS therapies are being evaluated, including Na + channel blockers, K + channel activators, alpha-adrenergic receptor blockers, protein-kinase inhibitors, and atropine. However, current experience with these drugs is limited.
LQT3.
Currently, LQT3 is the only LQTS variant for which a gene-specific therapy is recommended by current guidelines (see Table 31.9 ). Given the fact that augmentation of the late Na + inward current (I NaL ) underlies the prolongation of the QT interval in patients with the LQT3 genotype, Na + channel blockers have been successfully used in these patients. Mexiletine, a class IB Na + channel blocker, was shown to shorten the QT interval and reduce the occurrence of arrhythmic events in LQT3 patients.
Until prospective clinical trials confirm the effectiveness of mexiletine, it should be used in LQT3 patients with a QTc exceeding 500 milliseconds only in conjunction with beta-blockers or with the backup of an ICD. In addition, some investigators recommend testing the effectiveness of mexiletine by the administration of half the daily dose during continuous ECG monitoring. Only if the QTc is shortened by more than 40 milliseconds within 90 minutes of drug administration (when the peak plasma concentration is reached) should mexiletine be added to beta-blocker therapy.
Flecainide, a class IC Na + channel blocker, was shown to shorten the QT interval in LQT3 patients with a specific mutation (D1790G) in the SCN5A gene. However, flecainide is reported to elicit a Brugada phenotype in some LQT3 patients; therefore this drug should not be used in LQT3 patients except for those with this specific SCN5A mutation.
The antianginal agent ranolazine has unique EP properties. Unlike other Na + channel blockers, which reduce both the early (peak) and late components of the Na + current (I Na and I NaL , respectively), ranolazine preferentially reduces I NaL . Moreover, ranolazine reduces Ca 2+ overload of myocardial cells and suppresses EAD-triggered arrhythmias in animal models of LQT3. Ranolazine was shown to shorten the QT interval without widening the QRS complex in LQT3 patients, and can potentially offer a therapeutic benefit in these patients.
LQT2.
Potassium supplements can be of value, especially in LQT2 patients, who are particularly sensitive to low K + levels because the conductance of KCNH2 channels is directly related to extracellular K + concentrations. Therefore efforts should be made to maintain a serum K + level greater than 4 mEq/L in patients with this genotype. Acute treatment with IV potassium can be effective in suppressing torsades de pointes. Furthermore, long-term oral potassium supplements, even in patients with normal K + levels at baseline, can potentially reduce repolarization abnormalities in LQT2. Increasing extracellular K + concentrations enhances IKr, at least partially compensates for the loss of I Kr in LQT2, and can potentially limit the development of an arrhythmogenic substrate under long QT conditions. Whether these effects translate into clinical benefit in reduction of the risk of cardiac events remains to be proven.
Family Screening
Timely (often presymptomatic) identification of disease carriers is important because preventive measures and therapies can effectively avert SCD. Therefore when a patient is diagnosed with LQTS, ECGs should be obtained on all first-degree family members (i.e., parents, siblings, offspring) to determine whether others are affected. Unexplained sudden death in a young individual should trigger a similar evaluation to determine if LQTS is present in the family.
When the causal mutation has been identified in the proband, first-degree relatives should be offered genetic screening, even those with a negative clinical and ECG phenotype. Genotyping of family members can help exclude the diagnosis in some persons, and identify silent mutation carriers and allow prophylactic treatment. However, detailed genetic counseling is warranted before proceeding to this testing, particularly for asymptomatic persons for whom the option of not testing must also be recognized.
Long QT Syndrome
The LQTS is an uncommon inherited cardiac channelopathy that is associated with an abnormally prolonged QT interval and an increased propensity for life-threatening ventricular arrhythmias in the presence of a structurally normal heart.
In 1957, Anton Jervell and Fred Lange-Nielsen published the first report on a familial (autosomal recessive) disorder (“Jervell and Lange-Nielsen syndrome”) characterized by the presence of a striking prolongation of the QT interval, congenital deafness, and a high incidence of SCD at a young age. Subsequently, Romano and Ward independently identified an almost identical, but autosomal dominant, disorder that is not associated with deafness (“Romano-Ward syndrome”). A genetic relationship between the two was then proposed and the two syndromes were considered variants of one disease under the unifying name of “LQTS.” In the contemporary literature, Romano-Ward syndrome is used interchangeably with LQTS, but is now less commonly used in favor of the LQT1 to LQT17 scheme according to the underlying genetic mutation (see later).
The initial molecular studies suggested that all genes linked to the LQTS phenotype encode for various subunits of cardiac ion channels. Subsequent findings, however, revealed that LQTS could also be caused by mutations of gene coding for channel-associated cellular structural proteins as well. Nonetheless, the concept that LQTS genes ultimately affect cardiac ion currents, either directly (ion channel mutations) or indirectly (modulators), still holds true.
Genetics of the Long QT Syndrome
To date, more than 600 mutations of 17 different genes responsible for a hereditary form of LQTS have been identified (LQT1-17) ( Table 31.1 ; Fig. 31.1 ), with the majority (more than 90%) of the known mutations located in the first three genes: LQT1 (KCNQ1) mutations account for 40% to 55% of genetically positive LQTS, LQT2 (KCNH2) for 30% to 45%, and LQT3 (SCN5A) for 5% to 10%.
Disease | Gene | Protein | Functional Effect | Frequency |
---|---|---|---|---|
LQT1 | KCNQ1 (KvLQT1) | K v 7.1 | ↓I Ks | 40%–55% |
LQT2 | KCNH2 (HERG) | K v 11.1 | ↓I Kr | 30%–45% |
LQT3 | SCN5A | Na v 1.5 | ↑I Na | 5%–10% |
LQT4 (Ankyrin-B syndrome) | ANKB | Ankyrin-B | Aberrant ion channel/transporter localization | <1% |
LQT5 | KCNE1 | MinK | ↓I Ks | <1% |
LQT6 | KCNE2 | MiRP1 | ↓I Kr | <1% |
LQT7 (Andersen-Tawil syndrome) | KCNJ2 | Kir2.1 | ↓I K1 | <1% |
LQT8 (Timothy syndrome) | CACNA1C | Ca v 1.2 | ↑I CaL | <1% |
LQT9 | CAV3 | Caveolin 3 | ↑ I Na | <1% |
LQT10 | SCN4B | Na v β4 | ↑I Na | <1% |
LQT11 | AKAP9 | Yotiao | ↓I Ks | <1% |
LQT12 | SNTA1 | Syntrophin-α1 | ↑I Na | <1% |
LQT13 | KCNJ5 | Kir3.4 (GIRK4) | ↓I KACh | <1% |
LQT14 | CALM1 | Calmodulin 1 | ↑I CaL | 1%–2% |
LQT15 | CALM2 | Calmodulin 2 | ↑I CaL | <1% |
LQT16 | CALM3 | Calmodulin 3 | ↑I CaL | <1% |
LQT17 (Triadin knockout syndrome) | TRDN | Triadin | ↑I CaL | 2% |
JLN1 | KCNQ1 (KvLQT1) | K v 7.1 | ↓I Ks | Very rare |
JLN2 | KCNE1 | MinK | ↓I Ks | Very rare |

Overall, nine of these genes encode ion channel subunits that are specifically involved in cardiac action potential generation. LQT4, LQT9, LQT11, LQT12, and LQT14-LQT17 are caused by mutations in a family of versatile membrane adapters other than ion channel subunits.
The majority of LQTS cases are inherited in an autosomal dominant fashion. Conversely, Jervell and Lange-Nielsen syndrome, which is inherited in an autosomal recessive fashion, is very rare, affecting less than 1% of LQTS cases.
Genetic analysis reveals two or more mutations in 8% to 11% of LQTS patients with clinical phenotypes of autosomal dominant Romano-Ward syndrome. These compound mutations (so-called “double hits”) appear to be associated with a more severe phenotype than that associated with a single hit.
Most reported LQTS genetic mutations occur in coding regions, although noncoding mutations (resulting in the loss of allele expression) have also been described. Most LQTS families have their own mutations, which are often termed “private” mutations.
Several genetic mechanisms have been implicated in the development of LQTS including abnormalities in protein synthesis (transcription, translation), posttranslational protein processing resulting in abnormal transport to the cell surface membrane (protein trafficking, folding, assembly of subunits, glycosylation), ion channel gating (biophysical and kinetic properties), or permeation (ion selectivity, unitary conductance).
The majority of LQTS cases are caused by heterozygous disease; thus mutations causing abnormalities in channel coassembly or trafficking result in up to 50% maximal reduction in the number of functional channels (haplotype insufficiency), because the gene product from the healthy allele remains intact. On the other hand, mutations that abolish channel function while preserving subunit assembly can result in dominant-negative suppression of the healthy allele as well, causing a more severe reduction (up to 94%) of the total amount of functional protein (dominant-negative effect) and favoring a more severe clinical course and a higher frequency of arrhythmia-related cardiac events.
Mutations Related to the Potassium Current
Mutations related to the slowly activating delayed rectifier potassium current.
Slowly activating delayed rectifier potassium current (I Ks ) contributes to human atrial and ventricular repolarization, particularly during action potentials of long duration, and plays an important role in determining the rate-dependent shortening of the cardiac action potential. Mutations in LQT1, LQT5, and LQT11 result in attenuation of I Ks and, as a consequence, prolongation of repolarization, action potential duration and QT interval. LQT1 is caused by loss-of-function mutations of the KCNQ1 (KvLQT1) gene, which encodes the α subunit (K v 7.1) of the inward I Ks . More than 170 mutations of this gene have been reported, comprising many Romano-Ward (autosomal dominant) syndromes and accounting for approximately 40% to 55% of all genotyped LQT families. Of note, mutations involving the transmembrane domain of KCNQ1 result in more severe disease compared with C-terminal mutations. LQT5 is caused by loss-of-function mutations of the KCNE1 gene, which encodes the β- subunit (MinK) that modulates I Ks .
Homozygous or compound heterozygous loss-of-function mutations of either the KCNQ1 or KCNE1 genes cause the autosomal recessive form of LQTS (the Jervell and Lange-Nielsen syndrome). Patients with KCNQ1 mutations (type 1 Jervell and Lange-Nielsen syndrome) have an almost sixfold greater risk of arrhythmic events, whereas patients with KCNE1 mutations (type 2 Jervell and Lange-Nielsen syndrome) appear to be at lower risk. Although the Jervell and Lange-Nielsen syndrome is the most severe among the major variants of LQTS, the parents of Jervell and Lange-Nielsen syndrome patients are generally less symptomatic than other LQT1 patients, despite the fact that they all are heterozygous for the same gene. This is likely related to the observation that most of the LQT1 genetic variants are missense mutations exerting a dominant-negative effect, whereas most (74%) Jervell and Lange-Nielsen mutations of KCNQ1 are frame-shift/truncating mutations that are unable to cause dominant-negative suppression but are likely to interfere with subunit assembly. Jervell and Lange-Nielsen syndrome accompanies complete loss of I Ks in hair cells and endolymph of the inner ear, which results in congenital deafness.
LQT11 is caused by loss-of-function mutations of the AKAP9 gene, which encodes an A-kinase anchoring protein (Yotiao), shown to be an integral part of the I Ks macromolecular complex. The presence of Yotiao is necessary for the physiological response of the I Ks to beta-adrenergic stimulation. LQT11 mutations reduce the interaction between Yotiao and the I Ks channel (K v 7.1), preventing the functional response of I Ks to cyclic adenosine monophosphate (cAMP) and adrenergic stimulation and causing an attenuation of I Ks .
Mutations related to the rapidly activating delayed rectifier potassium current.
Rapidly activating delayed rectifier potassium current (I Kr ) presents the principal repolarizing current at the end of the plateau phase in most cardiac cells and plays an important role in governing the cardiac action potential duration and refractoriness. Mutations in LQT2 and LQT6 result in attenuation of I Kr and cause a decrease in the K + outward current and prolongation of repolarization, action potential duration, and QT interval.
LQT2 is caused by loss-of-function mutations of the KCNH2 (HERG) gene, which encodes the α-subunit (K v 11.1) of the inward I Kr . LQT2 syndrome accounts for almost 30% to 45% of all genotyped congenital LQTS cases. Approximately 200 mutations in this gene have been identified, which result in rapid closure of the hERG channels and decrease the normal rise in I Kr , leading to delayed ventricular repolarization and QT prolongation. Mutations involving the pore region of the hERG channel are associated with a significantly more severe clinical course than nonpore mutations; most pore mutations are missense mutations with a dominant-negative effect.
LQT6 is caused by loss-of-function mutations of the KCNE2 gene, which encodes the accessory β-subunit (MiRP1) of the hERG channel. LQT6 displays clinical resemblance to LQT2.
Mutations related to the inward rectifier potassium current.
Inward rectifier potassium current (I K1 ) contributes to the terminal portion of phase 3 repolarization. Andersen-Tawil syndrome (LQT7) is caused by loss-of-function mutations of the KCNJ2 gene, which encodes the voltage-dependent K + channel (Kir2.1) that contributes to the inward I K1 . Kir2.1 channels are expressed primarily in skeletal muscle, heart, and brain. The majority of mutations exert a dominant-negative effect on channel current.
Disruption of the I K1 function can potentially lead to prolongation of the terminal repolarization phase and QT interval, which can predispose to the generation of early afterdepolarizations (EADs) and delayed afterdepolarizations (DADs) causing ventricular arrhythmias. However, unlike other types of LQTS, where the afterdepolarizations arise from reactivation of L-type Ca 2+ channels, the EADs/DADs generated in LQT7 are likely secondary to Na + -Ca 2+ exchanger-driven depolarization. It is believed that the differential origin of the triggering beat is responsible for the observed discrepancy in arrhythmogenesis and the clinical features compared with other types of LQTS. In addition, it is likely that prolongation of the action potential duration in LQT7 is somewhat homogeneous across the ventricular wall (i.e., transmural dispersion of repolarization is less prominent than in other types of LQTS), which can potentially explain the low frequency of torsades de pointes. Flaccid paralysis results from failure to propagate action potentials in the skeletal muscle membrane as a result of sustained membrane depolarization.
Mutations related to the acetylcholine-activated potassium current.
LQT13 is caused by loss-of-function mutations of the KCNJ5 gene, which encodes the α subunit (Kir3.4, GIRK4) of the inward acetylcholine-activated potassium current (I KACh ). Kir3.4 mutations exert dominant-negative effects on Kir3.1/Kir3.4 channel complexes by disrupting membrane targeting and stability of Kir3.4.
Mutations Related to the Sodium Current
LQT3 is caused by gain-of-function mutations of the SCN5A gene, which encodes the α subunit (Na v 1.5) of the cardiac voltage-gated Na + channel that is responsible for the sodium content (I Na ). LQT3 accounts for approximately 5% to 10% of genotype-positive LQTS cases. More than 200 mutations have been identified in the SCN5A gene, with the majority being missense mutations mainly clustered in Na v 1.5 regions that are involved in fast inactivation, or in regions that stabilize fast inactivation.
Several mechanisms have been identified to underlie ionic effects of SCN5A mutations in LQT3. Most of the SCN5A mutations cause a gain of function through disruption of fast inactivation of the Na + channel, allowing repeated reopening during sustained depolarization and resulting in an abnormal, small but functionally important sustained (or persistent) noninactivating Na + current (I sus ) during the action potential plateau that acts to slow repolarization and prolong the action potential duration ( eFig. 31.1 ). Other, less common mechanisms ( eFig. 31.2 ) include increased window current, which results from delayed inactivation of mutant Na + channels, occurring at more positive potentials and widening the voltage range during which the Na + channel may reactivate without inactivation. In addition, some mutations cause slower inactivation, which allows longer channel openings, and causes a slowly inactivating Na + current (the late Na + current, I NaL ).


Regardless of the mechanism, increased Na + current (I sus , window current, I NaL , or peak I Na ) upsets the balance between depolarizing and repolarizing currents in favor of depolarization. Gain-of-function LQT3 mutations often increase I NaL two- to fourfold. Because the general membrane conductance is small during the action potential plateau, the presence of a persistent inward Na + current, even of small amplitude, can potentially have a major impact on the plateau duration and can be sufficient to prolong repolarization and QT interval. The resulting delay in the repolarization process triggers EADs (i.e., reactivation of the L-type Ca 2+ channel during phase 2 or 3 of the action potential), especially in Purkinje fiber myocytes where action potential durations are intrinsically longer. Also, increased influx of Na + (via the enhanced I NaL ) can stimulate the Na + -Ca 2+ exchanger in the reverse mode (3 Na + ions out, one Ca 2+ ion in), with consequent intracellular Ca 2+ overload and DADs. QT prolongation and the risk of developing arrhythmia is more pronounced at slow heart rates, when the action potential duration is longer, allowing more Na + current to enter the cell.
LQT9 is caused by gain-of-function mutations of the CAV3 gene, which encodes caveolin-3, a plasma membrane scaffolding protein that interacts with Na v 1.5 and plays a role in compartmentalization and regulation of channel function. Mutations in caveolin-3 induce kinetic alterations of the Na+ channel that result in persistent late Na + current (I sus ) and have been reported in cases of SIDS.
LQT10 is caused by gain-of-function mutations of the SCN4B gene, which encodes the β-subunit (Na v β4) of the Na v 1.5 ion channel. To date, only a single mutation in one patient has been described, which resulted in a shift in the inactivation of the Na + current toward more positive potentials, but did not change the activation. This resulted in increased window currents at membrane potentials corresponding to phase 3 of the action potential.
LQT12 is caused by mutations of the SNTA1 gene, which encodes α1-syntrophin, a cytoplasmic adaptor protein that enables the interaction between Na v 1.5, nitric oxide synthase, and sarcolemmal Ca 2+ adenosine triphosphatase (ATPase) complex that appears to regulate ion channel function. By disrupting the interaction between Na v 1.5 and sarcolemmal calcium ATPase complex, SNTA1 mutations cause increased Na v 1.5 nitrosylation with consequent reduction of channel inactivation and increased I sus densities.
Mutations Related to the Calcium Current
Mutations related to the L-type calcium current.
Timothy syndrome (LQT8) is caused by gain-of-function mutations of the CACNA1C gene, which encodes the α subunit (Ca v 1.2) of the voltage-dependent L-type Ca 2+ channel that contributes to L-type calcium current (I CaL ).
Recently, multiple nonsyndromic LQTS-causative mutations in CACNA1C were described in LQTS patients with isolated QT prolongation and propensity to ventricular arrhythmias in the absence of congenital heart defects and extracardiac manifestations that define Timothy syndrome clinically (nonsyndromic LQT8). Other CACNA1C mutations were found to cause cardiac-only Timothy syndrome (COTS), characterized by the concomitant but variably expressed phenotypes of LQTS (QT prolongation), hypertrophic cardiomyopathy, congenital heart defects, and SCD in the absence of any extracardiac symptoms.
The inward I CaL sustains depolarization and gives rise to the plateau phase essential for excitation-contraction coupling. Gain-of-function mutations of CACNA1C result in near complete elimination of voltage-dependent inactivation of Ca v 1.2 channels, leading to inappropriate continuation of the depolarizing I CaL and lengthening of the plateau phase, predisposing to the generation of EADs. In addition, augmentation of I CaL leads to intracellular Ca 2+ overload, which promotes spontaneous ectopic Ca 2+ release from sarcoplasmic reticulum and the generation of potentially arrhythmogenic DADs.
The exact pathophysiology of hypertrophic cardiomyopathy, congenital heart defects, and extracardiac manifestations of Timothy syndrome and their relation to CACNA1C mutations remain unclear.
Mutations related to calmodulin (calmodulinopathy).
Calmodulin is a small cytoplasmic Ca 2+ -binding protein with ubiquitous expression. Calmodulin is involved, directly and indirectly, in modulation of several ion channels, including Na + , K + , L-type Ca 2+ , and RYR2 channels. The main binding partner of calmodulin in cardiac cells is RyR2, which regulates Ca 2+ release from the sarcoplasmic reticulum. Acting as an intracellular Ca 2+ sensor, Ca 2+ -saturated calmodulin binds RYR2 to inhibit Ca 2+ release by stabilizing the RyR2 channel closed state.
Three different genes (CALM1–3) in the human genome encode exactly the same calmodulin protein. Recent genetic studies have identified several calmodulin mutations associated with CPVT, LQTS, and idiopathic VF. Defective calmodulin–RyR2 binding results in impaired calmodulin inhibition of RyR2 function with consequent dysregulation of sarcoplasmic reticulum Ca 2+ release. Further, de novo mutations in CALM1, CALM2, or CALM3 genes were found to disrupt Ca 2+ -dependent inactivation of the cardiac L-type Ca 2+ channel (Ca v 1.2), which leads in augmentation of I CaL , prolongation of the plateau phase of action potential, and LQTS phenotype (LQT14–16).
Mutations related to triadin: triadin knockout syndrome.
TRDN encodes triadin, a sarcoplasmic reticulum protein functionally and physically related to the RYR2. Homozygous or compound heterozygous loss-of-function mutations in TRDN likely reduce triadin-mediated negative feedback on the L-type Ca 2+ channel, resulting in augmentation of I CaL , prolonged action potential duration, and the recessively inherited LQTS phenotype (LQT17). Intracellular Ca 2+ overload and increases in spontaneous sarcoplasmic reticulum Ca 2+ release, particularly in the setting of beta-adrenergic stimulation, result in ventricular arrhythmias.
LQT17 is characterized by extensive T wave inversions in the precordial leads V 1 through V 4 , with either persistent or transient QT prolongation, exercise-induced cardiac arrest in early childhood (2 to 6 years of age), and mild-to-moderate proximal skeletal muscle weakness. Because all TRDN -null patients display a strikingly similar phenotype, it has been proposed that either triadin knockout syndrome or TRDN -mediated autosomal-recessive LQTS should be used rather than LQT17.
Mutations in the Ankyrin-B Gene: Ankyrin-B Syndrome
LQT4 is caused by loss-of-function mutations of the ANK2 gene, which encodes ankyrin-B, a structural membrane adapter protein that anchors ion channels to specific domains in the plasma membrane. Functionally, ankyrins bind to several ion channel proteins, targeting these proteins to specialized membrane domains, such as the anion exchanger (chloride-bicarbonate exchanger), Na + -K + ATPase, I Na , the Na + -Ca 2+ exchanger (I Na – Ca ), and Ca 2+ release channels (including those mediated by the receptors for inositol triphosphate [IP 3 ] or RyR2). Hence, ANK2 mutations can potentially result in improper localization and activity of ion-conducting proteins.
Mutations of the ANK2 gene have been reported to lead to increased intracellular concentration of Ca 2+ and, sometimes, fatal arrhythmia. However, QT interval prolongation is not a consistent feature in patients with ankyrin-B dysfunction, and the clinical phenotypes often extend beyond the typical LQTS, including sinus node dysfunction (SND), atrioventricular (AV) block, and atrial fibrillation (AF), in addition to idiopathic VF, exercise-induced, polymorphic VT, and SCD. Therefore ankyrin-B dysfunction is now regarded as a clinical entity distinct from classic LQTS (referred to as the “ankyrin-B syndrome”).
Pathophysiology of the Long QT Syndrome
Mechanism of QT Interval Prolongation
Any factor that evokes lengthening of the action potential duration holds the potential of causing an LQTS phenotype, especially if it does it heterogeneously. Electrophysiologically, prolongation of the action potential duration and QT interval can arise from either a decrease in the outward repolarizing current (K + currents: I Kr , I Ks , I K1 , I KACh ) or an increase in inward depolarizing membrane current (I Na , I CaL ) during phases 2 and 3 of the action potentials ( Fig. 31.2 ).
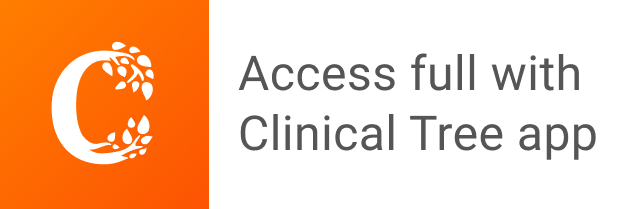