Structural heart disease
Reentry
Triggered activity
Automaticity
Infarct-related cardiomyopathy
Coronary artery disease/Myocardial infarction
++++
+
++
Non-ischemic cardiomyopathy
Dilated cardiomyopathy
++++
+
++
Hypertrophic cardiomyopathy
++++
+
+
Arrhythmogenic RV cardiomyopathy
++++
+
+
Heart failure
++++
++
+
Automaticity
Automaticity in cardiac myocytes may be normal or abnormal. Normal automaticity originates in cells with intrinsic pacemaker properties at baseline whereas abnormal automaticity occurs in cells that do not normally have automaticity but express it when membrane properties are altered by disease. Pathological environments lead to a reduction in resting membrane potential and when reduced sufficiently, spontaneous diastolic depolarization may occur and lead to impulse initiation [3]. Some cells, such as Purkinje system myocytes, can display normal and abnormal automaticity, expressing normal automaticity at high levels of membrane potential and abnormal automaticity when the membrane potential is reduced [3]. Ventricular myocytes on the other hand, only develop automaticity when they are partially depolarized due to underlying pathology and automaticity in ventricular myocytes is always abnormal. Under normal conditions, there is a hierarchy of intrinsic pacemaker rates with the sinus node being the most rapid, followed by the atria, the AV node, the His bundle, and the Purkinje fibers respectively. In this hierarchy slower latent pacemaker cells are depolarized by propagating wavefronts originating from faster pacemaker cells preventing them from reaching their own spontaneous depolarization threshold potential. These latent pacemaker cells are inhibited by repeated depolarization, a phenomenon known as overdrive suppression [3, 4]. For automatic VT to occur, the pacemaker rate in the Purkinje cells or ventricular myocytes must increase above that of the sinus node and higher pacemaker cells. The most important cause of this is sympathetic activation, which enhances the rate of spontaneous diastolic depolarization in latent ventricular pacemakers more than the sinus node. Catecholamine stimulation is generally required for the development of automatic ventricular arrhythmias.
Triggered Activity
Triggered activity describes impulse initiation dependent on after-depolarizations, which are oscillations in membrane potential that follow the upstroke of an action potential. There are two kinds of after-depolarizations. One occurs early during repolarization (early after-depolarizations (EADs)) and the other is delayed until repolarization is complete or nearly complete (delayed after-depolarizations (DADs)) [5, 6]. A triggered impulse is initiated when an after-depolarization depolarizes a cell to its threshold potential leading to initiation of an action potential. A triggered action potential can then be followed by another after-depolarization that may or may not reach threshold. When it does reach threshold, a “train” of additional triggered action potentials can occur each arising from the after-potential caused by the previous action potential.
DADs occur when Ca2+ in the myoplasm and sarcoplasmic reticulum (SR) increases above normal levels (“calcium overload”). Abnormalities in the sequestration and release of Ca2+ by the SR may also contribute to their occurrence. When intracellular Ca2+ is elevated, Ca2+ in the SR may rise during repolarization to a critical level at which time a secondary, spontaneous release of Ca2+ occurs after the action potential generating a transient inward current (the DAD) [3, 5, 6]. This increase in intracellular Ca2+ may be a result of increase in heart rate or premature stimulation, digitalis inhibition of Na+/K+ pump, catecholamine enhanced L-type Ca2+ current, or other effects of pathology that increase Ca2+ loading such as heart failure or hypertrophy [3, 5, 6].
As opposed to DADs, EADs occur when repolarization of the action potential does not follow the normal smooth trajectory but suddenly shifts in a depolarizing direction. This occurs when outward current slows or inward current increases, at least transiently. When the relationship between inward and outward current is altered such that depolarization reaches threshold, inward current can be reactivated causing an action potential upstroke [5, 7, 8]. EADS occur under conditions that delay repolarization resulting in prolongation of the action potential duration, either by increasing inward current or decreasing outward current. They occur more readily in Purkinje fibers than in ventricular or atrial muscle. Antiarrhythmic drugs that prolong the duration of the action potential of Purkinje fibers (i.e. class III antiarrhythmic drugs (AADs) such as sotalol and dofetilide or class IA AADs such as quinidine) can cause EADs through inhibition of the IKr repolarizing current [9]. Hypoxia, the combination of hypoxia and acidosis with or without catecholamines, and stretch are other causes of EADs to name a few [3, 5]. All causes of EADs are manifest on the surface electrocardiogram (ECG) as QT prolongation. EADs are the trigger of functional reentrant excitation leading to polymorphic VT.
Slow Conduction and Reentry
Reentrant excitation requires a region of unidirectional block, at least transiently, allowing for propagation of an impulse in one direction while preventing excitation in the other direction over the return pathway, which the impulse eventually uses to reenter the region and re-excite. Transient block can occur after rapid repetitive activation or after premature excitation. Reentry also may occur when there is permanent unidirectional block. In addition, for reentry to recur, the impulse conducting through the reentrant pathway must find excitable tissue. This requires that conduction time around the circuit is longer than the effective refractory period of the myocardium from which it originated. Slow conduction and/or short refractory periods permit reentry to occur and are often a consequence of underlying pathology. The reentrant circuit can have an anatomical component with a central obstacle or it may be functional with its size and shape determined by electrophysiologic properties of the involved tissue.
Myocardial fibrosis and scarring in a diseased heart affects conduction by several mechanisms. It increases the extracellular resistivity of myocyte bundles leading to slow conduction and block. Fibrosis can also distort the size and shape of myocardial bundles trapped in scar tissue leading to changes in their conductive properties. Changes in cell-to-cell connections caused by fibrosis can also lead to conduction abnormalities in the diseased heart. During normal conduction of an impulse, axial current flows from one myocardial cell to another through gap junctions [10]. Gap junctions are specialized regions of close interaction between neighboring myocytes in which clusters of transmembrane channels bridge the paired plasma membranes [11]. Gap junctions are mainly located at the ends of myocytes in the intercalated disks and provide low resistance pathways for current flow between cells, which is important for impulse propagation. The primary proteins making up the channels of gap junctions are called connexins. Connexin 43 is the primary connexin found in the ventricles [12, 13] whereas connexin 40 is primarily found in the His-Purkinje system (HPS). The orientation of gap junctions leads to more rapid conduction through the ventricular myocardium in the longitudinal direction, a property termed anisotropic conduction [14, 15]. This property can be altered by fibrosis and remodeling of gap junctions, which may predispose a diseased heart to slow conduction, unidirectional block, and reentry. An increased resistance to axial current flow caused by pathological structural alterations in gap junctions decreases the magnitude and spread of current along a myocardial bundle which can decrease conduction velocity and cause conduction block. The most important causes of slow conduction and reentry are connexin dysfunction and fibrosis, which often coexist (Fig. 15.1).


Fig. 15.1
Myocardial Structural Remodeling Promotes Conditions for Reentry. Structural heart disease in heart failure and cardiomyopathy leads to pathophysiologic changes which result in abnormal impulse formation and propagation setting the stage for reentrant excitation. Abnormalities in cell-to-cell connectivity, extracellular resistance, and ion channel remodeling all contribute to the increased likelihood of reentrant arrhythmias in heart failure and cardiomyopathy. See text for discussion
Heart Failure and Arrhythmogenesis
As previously mentioned, all of the classic mechanisms of arrhythmogenesis may play a role in the development of ventricular arrhythmias in heart failure. Fibrosis and scarring of the ventricles due to myocardial infarction (MI), pathologic hypertrophy, non-infarct related fibrosis, or myocardial dysplasia predispose patients with cardiomyopathy to reentrant ventricular arrhythmias. Heart failure is associated with changes in the cardiac ion channel currents that lead to abnormal depolarization and repolarization, predisposing to automatic and triggered activity. These changes are at least in part due to the neurohormonal activation and biomechanical abnormalities associated with the heart failure syndrome (Table 15.2).
Table 15.2
Components of the arrhythmogenic substrate in structural heart disease
Structural heart disease | Fibrosis | Myocyte hypertrophy | Non-uniform anisotropy | Gap junction remodeling | Neural factors | Ion channel changes |
---|---|---|---|---|---|---|
Infarct-related cardiomyopathy | ||||||
Coronary artery disease/Myocardial infarction | +++ | +++ | +++ | +++ | +++ | +++ |
Non-ischemic cardiomyopathy | ||||||
Dilated cardiomyopathy | +++ | +++ | ? (likely) | +++ | +++ | ?? |
Hypertrophic cardiomyopathy | +++ | +++ | ? (likely) | +++ | ?? | ?? |
Arrhythmogenic RV cardiomyopathy | +++ | +++ | ? (likely) | +++ | ?? | ?? |
Heart failure | +++ | +++ | ? (likely) | +++ | +++ | +++ |
Fibrosis
Fibrosis is the most common cause of functional and anatomic block leading to slow conduction and reentry and occurs in both infarct-related and nonischemic cardiomyopathies. The pattern and extent of fibrosis may range from patchy areas in some forms of heart failure to extensive fibrotic scars in MI. In infarct-related cardiomyopathies, fibrosis replaces necrotic regions and may extend into non-infarcted bordering regions. The number of cell-to-cell contacts is reduced with side-to-side connections selectively affected [16, 17]. In healing infarct border zones, increased interstitial collagen disrupts the long transversely oriented gap junctions enhancing anisotropy by increasing the axial resistivity transverse to the long fiber axis [17]. Gap junctions at the ends of myocytes undergo fewer changes. Thus the normal property of uniform anisotropy is converted to non-uniform anisotropy [16, 17].
When there is a large scar such as in hearts with myocardial infarcts, “peninsulas” and bridges of intact myocytes can project into or completely across areas of fibrosis termed “channels”. Myocytes in these bridges sometimes link with normal myocytes on the outer borders of the infarct [18]. Slow conduction can result from circuitous, nonuniform propagation through these myocardial bundles trapped in scar tissue, so-called “zig-zag conduction” [19]. The effects of fibrosis on conduction is often detectable by low amplitude, long duration, fractionated electrograms with delayed activation, the basis for substrate mapping for ablation of VT [20]. The number of fractionated electrograms seen during endocardial substrate mapping has been shown to correlate with the likelihood of inducible monomorphic ventricular tachycardia at the time of electrophysiology study [21]. Fractionated electrograms are also more likely to be seen during endocardial mapping in patients with infarct-related and nonischemic cardiomyopathy who present with VT when compared to patients with no VT, nonsustained ventricular tachycardia (NSVT), or cardiac arrest [22].
Ischemia
Acute ischemia may result in abnormal automaticity or focal discharges from calcium overload and triggered activity in the form of delayed or early after-depolarizations [23, 24]. Acute ischemia activates the ATP-sensitive potassium (KATP) channels, causing an increase in extracellular potassium in the cardiac muscle. Minor increases in extracellular potassium depolarize the myocyte’s resting membrane potential, which can increase tissue excitability in early phases of ischemia [25]. Further hyperkalemia causes greater resting depolarization, decreased conduction velocity and tissue excitability, and shortening of the action potential duration, but prolongs the effective refractory period due to post-repolarization refractoriness [25]. These changes provide a substrate for injury current to flow between ischemic and nonischemic cells located in border zones, which can promote focal abnormal automaticity and initiate VT [26, 27].
Action Potential Prolongation and Abnormal Calcium Handling
Prolongation of the action potential has been repeatedly demonstrated in isolated myocytes [28] and intact ventricular preparations [29] from failing hearts independent of the cause. Downregulation of the transient outward current (Ito) is the most consistent ionic current change observed in heart failure [30–32] but downregulation of other potassium channel currents including IK1, IKr, and IKs have also been reported in several models of heart failure [30, 33–37]. Another mechanism for altered repolarization is a late sodium current, found in high density in myocytes of animals with chronic heart failure [38].
Alterations in Ca2+ handling are also involved in action potential prolongation and a predisposition to ventricular arrhythmias in heart failure. Abnormal intracellular calcium handling is a prominent feature of myocytes from failing hearts [39, 40]. Variable changes in the Na+/Ca+ exchanger have been reported with the majority of studies reporting an increase in function [41]. Downregulation of SERCA is also a consistent finding across studies [42–44] as is an increased open probability of the ryanodine receptor (the calcium release channel of the SR) [45, 46]. The basis for delayed afterdepolarizations is spontaneous calcium release from the SR which is facilitated by the presence of beta-adrenergic stimulation and the increased open probability of the ryanodine receptor [47]. Several studies have shown that adrenergic stimulation is necessary for the eliciting spontaneous calcium release from the SR and delayed afterdepolarizations [47, 48]. The calcium released is removed from the cell by the electrogenic Na+/Ca2+ exchanger whose function is upregulated in heart failure. This leads to a transient inward Na+ current that causes the delayed after-depolarization [49]. This is facilitated by the higher resting membrane potential created by a decrease in outward potassium current.
As previously mentioned, Purkinje myocytes are commonly the sources of afterdepolarizations associated with triggered arrhythmias and these cells undergo substantial remodeling of both K+ and Ca2+ currents, prolonging the action potential and leading to labile repolarization in these cells [50]. Prolongation of the action potential duration associated with down regulation of repolarizing currents and an increase in depolarizing currents lead to spatially and temporally labile repolarization that can predispose to after-depolarization-mediated triggered activity and functional reentry. The existence of after-depolarizations and reentry are not mutually exclusive. After-depolarizations that are of sufficient amplitude to illicit an action potential may serve to initiate an arrhythmia while reentry may sustain such arrhythmias [2].
Mechanical Factors
Mechanical abnormalities in patients with heart failure such as increased wall stress and cavitary dilatation can alter the electrophysiologic properties of myocardial tissue, termed mechanoelectric feedback [51, 52]. In the normal heart, acute myocardial stretch exaggerates the normal rate-dependent shortening of refractoriness but does not influence transverse or longitudinal conduction velocity [53]. In contrast, an animal model found that the development of dilated cardiomyopathy resulted in significant prolongation of refractoriness and repolarization that increased further by volume augmentation and was not reversed by pharmacologic load reduction [54]. Stretch has also been shown to cause a reduction of connexin 43 as well as its redistribution to the lateral sarcolemmal membranes resulting in slowing of conduction in the transverse direction, predisposing these cells to reentry [55]. In human studies, direct correlations between left ventricular end-diastolic volume and the prevalence of ventricular arrhythmia have been seen [56]. It has also been reported that the typical QT prolongation seen in patients with advanced heart failure can be significantly reversed by mechanically unloading the left ventricle with a left ventricular assist device [57].
Altered Neurohormonal Signaling
Activation of the adrenergic and renin-angiotensin-aldosterone system (RAAS) is known to be important in the progression of heart failure. Inhibition of these pathways in randomized clinical trials has been associated with reduction in overall mortality as well as SCD mortality [58–64]. Heart failure is associated with enhancement in sympathetic activity and a reduction in parasympathetic activity [65, 66]. This neurohormonal activation likely influences the substrate and triggers for ventricular arrhythmias in heart failure. In ventricular biopsies and autopsy specimens, an increased density and exaggerated spatial heterogeneity of sympathetic nerves have been associated with a previous history of ventricular arrhythmias in cardiomyopathies [67]. In the normal ventricle, sympathetic stimulation shortens the action potential duration and reduces the dispersion of repolarization, both associated with a decrease in arrhythmia potential [68]. However, in heart failure, sympathetic stimulation is a potent stimulus for the generation of arrhythmias perhaps by enhancing the dispersion of repolarization. Beta-adrenergic stimulation is known to have significant effects on calcium handling as noted above [2]. Elevated levels of sympathetic stimulation enhance the rate of spontaneous diastolic depolarization in latent ventricular pacemakers more than the sinus node which can lead to the development of automatic arrhythmias. Increases in intrinsic heart rate in the setting of sympathetic stimulation can also increase the likelihood of occurrence of triggered arrhythmias [6]. Furthermore, catecholamines can alter conduction and refractoriness, which may promote functional block and facilitate reentry.
RAAS signaling has numerous effects on the cardiovascular system that increase heart failure patients’ propensity to develop ventricular arrhythmias. The two major effectors of the RAAS axis, angiotensin II and aldosterone, are upregulated in heart failure and have prominent effects on properties of myocardial cells [2]. Angiotensin II can indirectly promote arrhythmia formation via potassium and magnesium loss in the urine resulting in prolongation of repolarization. It can also potentiate the effects of the sympathetic nervous system. Vasoconstriction caused by RAAS activation alters loading conditions, affecting wall stress and mechanical factors. Angiotensin II and aldosterone also promote generation of fibrosis in the myocardium by myofibroblasts [69]. Angiotensin II has also been shown to influence gap junction coupling in cardiac cells [70] possibly related to abnormal phosphorylation of connexin 43 [71] leading to nonuniform anisotropy and promoting reentry. Angiotensin II can also inhibit a number of K+ currents including the transient outward K+ current (Ito) and delayed rectifier K+ currents (IKr) in the myocardium [72–75].
Conduction System Disease and Bundle Branch Reentry
The QRS prolongation that often accompanies progression of myocardial disease can predispose patients to a unique arrhythmia called bundle branch reentrant ventricular tachycardia (BBRVT) [76, 77]. The unique properties of the HPS allow for rapid conduction and long refractory periods ordinarily preventing sustained reentry. However, conditions that result in prolongation of conduction in the HPS such as cardiomyopathy can facilitate sustained reentry [78]. Three circuits have been described in BBRVT. The first utilizes anterograde conduction over the right bundle branch and retrograde conduction over the left bundle branch with the His bundle adjacent to but separate from the circuit. The second form utilizes the left bundle as its anterograde limb and the right bundle for retrograde conduction. In more rare circumstances, intrafasicular reentry can also occur with the separate fascicles of the left bundle branch being used for the reentrant circuit [79]. Most patients that develop BBRVT tend to have advanced structural heart disease and QRS prolongation in the form of bundle branch block or nonspecific conduction delay. Although most have a baseline bundle branch block, the QRS prolongation in these patients is generally due to delayed conduction in one or both of the bundle branches rather than true block as complete block would not allow propagation of wavefronts retrogradely, which is required to facilitate reentry. This delay tends to be a result of the severity of the underlying heart disease with cardiac enlargement and heart failure being common.
Ventricular Arrhythmias and Heart Failure Etiology
The management and prognosis of ventricular arrhythmias vary based on the etiology of the underlying cardiomyopathy as well as the characteristics of the clinical arrhythmia. Although certain types of cardiomyopathy may predispose patients to the development of certain types of arrhythmias, all mechanisms of arrhythmogenesis can exist in all forms of heart failure. For example, sustained monomorphic VT due to reentry is common in infarct-related cardiomyopathy. However, such patients may also have tachycardias due to triggered activity from sites more classically associated with idiopathic VT such as the outflow tracts, fascicles, and coronary cusps. Similarly, certain cardiomyopathies may have a predisposition towards the development of arrhythmias originating in certain anatomic areas, such as the right ventricular inflow and outflow tracts in arrhythmogenic right ventricular cardiomyopathy. However, arrhythmias may originate from areas outside of these more classic sites in the same patients.
Infarct-Related Cardiomyopathy
Sustained monomorphic VT occurs most frequently in the setting of a healed MI and may occur in the subacute phase or long after the acute ischemic injury [80]. The extent of myocardial necrosis and subsequent fibrosis as well as the degree of left ventricular dysfunction are important determinants of arrhythmia risk following an MI. The overall incidence of sustained VT following an MI was classically reported to be between 3 and 5 percent but has been estimated to have declined to 1 % in recent years due to advances in revascularization resulting in smaller infarct scars [80]. Although most VTs in infarct-related cardiomyopathy are sustained through a reentrant mechanism, focal activation by abnormal automaticity or triggered activity from the ischemic border zone may serve to initiate the tachycardia, especially in the setting of ischemia. Reentry is the mechanism underlying VT associated with healed or healing MIs in more than 95 % of cases [80].
The 12-lead ECG during VT can provide important information regarding the presence or absence of prior myocardial infarction and localization of the VT origin. In the setting of prior MI, VT tends to arise subendocardially in the region of myocardial scarring. This results in qR or QR patterns of the QRS complexes overlying areas of infarction reflecting terminal activation of the subepicardium. A QS pattern can also occur in the setting of a prior full thickness MI. However, in this setting, the QS pattern represents a cavity potential and is of no value in localizing the VT origin. In the absence of a prior MI, such as in nonischemic cardiomyopathy, a QS pattern of the QRS complex may suggest an epicardial origin of the VT (Fig. 15.2).


Fig. 15.2
The 12-lead ECG of VT in the presence and absence of prior myocardial infarction. Panel (a) is the ECG of a monomorphic VT in a patient with a prior anteroseptal MI. The QRS complexes in the anterior precordial leads demonstrate a qR pattern in a distribution consistent with the previous anteroseptal MI representing terminal activation of the subepicardium in this region. Panel (b) is the ECG of a monomorphic ventricular tachycardia in a patient with nonischemic cardiomyopathy and no history of prior MI. None of the QRS complexes demonstrate a qR pattern consistent with the nonischemic substrate. The QS pattern seen in the lateral leads suggests an epicardial origin of the VT given the absence of prior infarction. See text for discussion
Although several possible anatomic abnormalities allowing for reentry in the setting of MI have been postulated, it is now accepted that reentry in the presence of MI utilizes surviving bundles of myocardium within the scar, separated by connective tissue, fibrosis, and disordered intercellular coupling [81] (Fig. 15.3). Evidence for this hypothesis includes the fact that fixed areas of slow conduction can be mapped during sinus rhythm in patients with VT and ablation at these sites can effectively eliminate VT [80]. The triggers for initiation of VT in this setting are increased in situations such as acute heart failure decompensation, which lead to surges in autonomic tone, electrolyte imbalances, and acute ischemia [24, 80].


Fig. 15.3
The Anatomic substrate for fractionated electrograms in coronary artery disease (CAD). Most ventricular tachycardias in the setting of prior myocardial infarction are sustained through a reentrant mechanism. Reentry is facilitated by slow conduction through surviving myocardial bundles trapped inside fibrotic myocardium. This slow conduction is detectable by low amplitude fractionated and late potentials with delayed activation represented above, left. Above right, fibrosis and scar from a prior myocardial infarction is pictured at the microscopic level. See text for discussion
Dilated Cardiomyopathy
The pathophysiologic basis for ventricular arrhythmias in dilated cardiomyopathies is not well understood although several possible mechanisms have been postulated [80]. Extensive myocardial abnormalities, fibrosis, and the loss of cell-to-cell coupling in patients with dilated cardiomyopathy may provide the substrate for reentry. Increased levels of fibrosis in the endocardium as well as the epicardium lead to increase in tissue anisotropy, particularly from epicardium to endocardium [82]. Most endocardial scars in dilated cardiomyopathy tend to be adjacent to the valve annulus while epicardial scars in these patients are often greater in extent than on the endocardium [83]. These epicardial scars may facilitate reentry due to development of conduction block in lines parallel to the epicardial fiber orientation between the epicardium and endocardium [82]. Focal mechanisms may also contribute to the genesis of ventricular arrhythmias in these patients. Spontaneous and induced VT in patients with dilated cardiomyopathy have been found to arise in the subendocardium through triggered activity from early or delayed after-depolarizations without evidence of reentry [84]. Patients with dilated cardiomyopathy have been shown to have nonhomogeneous distribution of sympathetic fibers. Regions with dense fibrosis show myocardial denervation similar to what is seen in a scar due to MI while other regions may show an increase in sympathetic innervations [85]. It is postulated that denervation hypersensitivity could lead to a regional increase in sensitivity to catecholamines. Patients with severe heart failure requiring transplantation have been shown to have regional increases in sympathetic nerves around diseased myocardium and blood vessels and this was shown to be more pronounced in patients with a history of VT [67]. Autoantibodies against beta-1 adrenergic receptors have also been detected in up to 50 percent of patients with dilated cardiomyopathy, some subgroups of which can exert sympathomimetic activity [86]. These autoantibodies have been associated with increased rates of ventricular arrhythmias in heart failure patients as well [86, 87]. It is important to note that patients with dilated cardiomyopathy are ten times more likely to develop cardiac arrest associated with polymorphic VT or ventricular fibrillation (VF) than to develop hemodynamically tolerated sustained monomorphic VT and this is likely an underestimate as this experience only includes survivors or cardiac arrest [80].
Hypertrophic Cardiomyopathy
Multiple variations of hypertrophic cardiomyopathy (HCM) exist with different clinical manifestations depending on the site and extent of hypertrophy. The hemodynamic consequences of myocardial hypertrophy in these patients include left ventricular outflow tract obstruction, mitral regurgitation, diastolic dysfunction, and myocardial ischemia. These patients have an increased likelihood of developing ventricular arrhythmias. Similar to all other etiologies of heart failure, it is thought that myocardial fibrosis, potentially related to ischemic damage, plays an important role in the arrhythmogenic substrate of HCM [88]. Late gadolinium enhancement (LGE) on cardiovascular magnetic resonance (CMR) imaging is a common finding in HCM patients, likely reflecting collagen deposition and fibrosis [89–91]. The extent of LGE in HCM patients has been correlated with SCD risk factors [90] as well as frequency of ventricular premature depolarizations (VPDs) and NSVT on ambulatory monitoring [92]. Although sustained monomorphic VT may occur in the presence of HCM, similar to dilated cardiomyopathies, these patients more commonly have cardiac arrest due to polymorphic VT or VF [80].
Arrhythmogenic Right Ventricular Cardiomyopathy
Arrhythmogenic right ventricular cardiomyopathy (ARVC) is characterized by fibrous or fibro-fatty replacement of the right ventricular myocardium in the inflow tract, outflow tract, or apex of the right ventricle. The right ventricular myocardial scarring initially produces regional wall motion abnormalities but as the disease progresses, it may involve the free wall and become global with right ventricular dilatation [93]. In some cases the left ventricle may also be involved [94]. These areas of scarring tend to be the focus of ventricular arrhythmias in patients with ARVC who often present with sustained or nonsustained monomorphic VT originating in the right ventricle. VTs originate from areas where scarring develops, most commonly the inflow tract, apex, or outflow tract of the right ventricle. As in the case with dilated cardiomyopathy, the fibrosis and scarring in this setting tends to be more pronounced in the epicardium than the endocardium. For individuals with advanced fibrosis, VT tends to be due to macroreentry in areas of epicardial scar. However, in patients with earlier stages of disease, VT may be due to triggered or automatic activity in the diseased myocardium, often related to adrenergic stimulation. In such instances, VT and SCD are often exercise-induced [95]. Exercise is thought to increase the risk of sudden death in ARVC patients, potentially due to catecholamine effects on triggered or automatic activity or due to the increased stress placed on the right ventricle during exercise [96]. Animal models of ARVC have suggested that exercise increases right ventricular dilatation and worsens manifestations of the disease [97]. In regards to the relationship of catecholamines and VT in patients with ARVC, a higher frequency of induced ventricular arrhythmias in patients with ARVC on isoproterenol infusion has been reported when compared to control patients [98]. Onset of VT has also been shown to be associated with gradual rises in sinus rates and shortening of coupling intervals prior to initiation suggesting an increase in sympathetic tone may play a role in initiation [99].
Ventricular Arrhythmias and Risk of Sudden Death
Ventricular arrhythmias in heart failure patients may range from VPDs to VF leading to SCD. VPDs occur in 70 to 95 percent of patients with heart failure [100–102]. The significance of these beats varies according to the etiology of the cardiomyopathy. VPDs in a patient with prior MI are associated with an increased risk of death. However, in patients with non-infarct-related cardiomyopathy, VPDs have not been uniformly shown to be associated with a worse prognosis [103]. The use of AADs to suppress VPDs has been shown to be harmful regardless of the etiology of heart failure due to their proarrhythmic effects [104, 105]. On the other hand, beta-blockers can often suppress VPDs and are indicated for most forms of heart failure regardless of the presence or absence of arrhythmias. In rare cases, very frequent VPDs may cause or worsen left ventricular dysfunction or patients may be highly symptomatic despite beta blockade. In such circumstances, treatment with AADs and catheter ablation is often necessary and can improve left ventricular function [106, 107]. When AADs are necessary, amiodarone has been shown to be safe in heart failure patients but it is not used for the routine suppression of ventricular arrhythmias as it has not been shown to provide any survival benefit [108, 109].
NSVT has been reported in 50 to 80 percent of heart failure patients on ambulatory monitoring [101, 110, 111]. The significance of NSVT in heart failure is similar to that of VPDs. In patients with infarct-related cardiomyopathy [112, 113] and hypertrophic cardiomyopathy [114–116], NSVT has been associated with increased mortality. However, this has not been reliably shown in other forms of heart failure such as dilated cardiomyopathy [101, 111, 117] and heart failure related to valvular disease [118]. As is the case with VPDs, there is no clear role for pharmacologic suppression of NSVT to reduce the risk of SCD, but NSVT can be an indication for electrophysiology study in order to better risk stratify heart failure patients with the hopes of determining who may benefit from implantable cardioverter-defibrillator (ICD) therapy [119, 120]. Like VPDs, in rare cases, very frequent NSVT can contribute to or exacerbate left ventricular dysfunction and may require more aggressive therapies such as AADs or catheter ablation.
Sustained VT is far less frequent than VPDs and NSVT in heart failure patients. Several studies have reported an incidence of sustained VT in patients with heart failure or cardiomyopathy of 5 % or less [101, 110, 111]. However, VT and VF cause the majority of sudden deaths in heart failure patients [1]. It is notable that all types of ventricular arrhythmias, including VPDs, NSVT, and sustained VT have been associated with increased mortality for most groups of heart failure patients. Despite the increased mortality associated with ventricular arrhythmias in heart failure, the rates of sudden cardiac death are not increased. Even as total mortality rises, the percentage of patients dying suddenly remains the same across groups. Approximately half of all deaths in heart failure patients are sudden, regardless of the amount of ventricular ectopy. For any given patient, the greater the severity of the heart failure, the more likely they are to die suddenly, irrespective to their history of ventricular arrhythmias.
Effect of Heart Failure Medications
Multiple classes of medications have been shown to improve mortality in heart failure patients due to systolic dysfunction. Standard therapy includes beta-blockers, ace inhibitors, angiotensin receptor blockers (ARBs), and aldosterone antagonists. Beta-blockers have been shown in multiple trials to impart a survival benefit to heart failure patients, mainly due to a significant reduction in SCD [61, 63, 121]. As previously discussed, heart failure is associated with enhancement of sympathetic activity and a reduction in parasympathetic activity [65, 66]. An increase in sinus rates in heart failure patients may be associated with an increased risk of sudden death, most likely due to an excess of sympathetic activity [2]. This may be because rapid rates increase the likelihood of triggered arrhythmias [6]. Although heart rate reduction has been associated with a survival benefit in heart failure patients, for any given reduction in heart rate and any baseline heart rate, beta blockade has been shown to further improve survival compared to placebo. This suggests that heart rate reduction is not the only mechanism responsible for the beneficial effects of beta blocker therapy [61].
Ace inhibitors and ARBs have been shown to improve all cause mortality in heart failure. However, their effects on SCD are less clear. Several major trials found that the survival benefit was primarily due to slowed progression of heart failure with little or no reduction in sudden death [60, 122, 123]. However, other large trials have found ace inhibitors and ARBs to be associated with significant reductions in sudden death [124–126]. Less controversial is the effect of aldosterone antagonists on sudden death. Both spironolactone and eplerenone have been shown to significantly reduce overall mortality and sudden death in patients with advanced heart failure [59, 127]. Spironolactone has also been shown to reduce the frequency of VPDs and NSVT [128]. The mechanisms by which modulation of the RAAS axis might decrease the risk of sudden death is not well understood. However, possible effects may include reverse remodeling of the arrhythmogenic substrate, decreased proliferation of fibrosis, and fewer effects of angiotensin II and aldosterone on active membrane properties [2]. Ace inhibitors and ARBs have been shown to prevent myocyte uncoupling and improve gap junction conductance through modulation of connexin 43 which may decrease the likelihood of sustained reentrant arrhythmias [55, 71]. Ace inhibitors, ARBs, and aldosterone antagonists may also decrease risk of ventricular arrhythmias through maintenance of a higher serum potassium concentration and decreased prolongation of action potential duration.
Therapies for Ventricular Arrhythmias
Ventricular arrhythmias are of greatest concern in heart failure patients because of the risks of developing a fatal arrhythmia leading to SCD. Although heart failure patients represent a high-risk group of patients for SCD, only a minority of patients with heart failure develops fatal ventricular arrhythmias. Knowing this, antiarrhythmic medications and ICDs that prevent or abort SCD will benefit some patients but most high-risk patients will never require these interventions [129]. Interventions that prevent or abort SCD carry risks. Multiple large randomized controlled trials have demonstrated that antiarrhythmic drugs have significant and often fatal proarrhythmic effects [104, 130]. ICDs have risks as well including infectious complications, inappropriate ICD discharges, and device malfunctions [131]. Furthermore, ICDs may have proarrhythmic effects [132]. Further advances in risk stratification of heart failure patients will be an important role of future research.
Antiarrhythmic Drug Therapy
The role of AADs for ventricular arrhythmias in patients with heart failure is limited. The use of AADs for suppression of ventricular arrhythmias fell out of favor after the CAST trial demonstrated that AADs had significant, often proarrhythmic effects [104]. This was later confirmed in large randomized trials, suggesting AADs might harm more patients than they benefit. Beyond their proarrhythmic effects, most antiarrhythmic drugs also have some negative inotropic activity, which can produce heart failure in patients with ventricular dysfunction [133]. Low cardiac output and the associated renal dysfunction that is common in heart failure can impair elimination of these drugs, increasing the risk of drug toxicity. Some of the AADs, particularly the class I and class III agents exert a strong proarrhythmic effect. Heart failure is a risk factor for the development of torsades de pointes in patients receiving the class III agents ibutilide, sotalol, and dofetilide. Amiodarone is generally considered to be less proarrhythmic than other AADs and is often the preferred drug for treatment of ventricular arrhythmias in heart failure. Amiodarone trials have shown mixed results on mortality and SCD [120, 134–136]. The 2005 ACC/AHA guidelines on the management of chronic heart failure do not recommend amiodarone for the prevention of SCD but concluded that the evidence was in favor of efficacy for its use for reducing the frequency of ICD shocks in patients with recurrent ventricular arrhythmias [137].
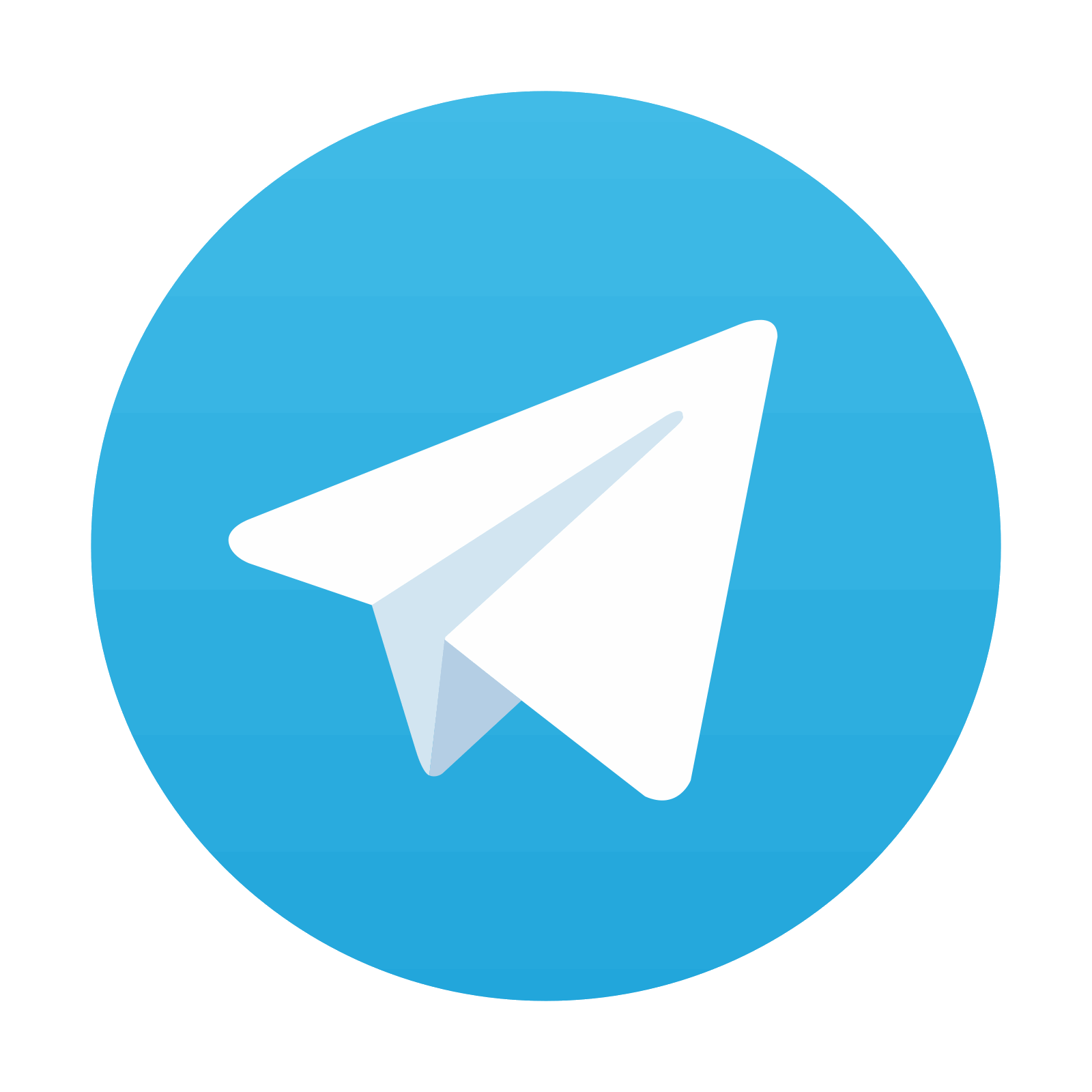
Stay updated, free articles. Join our Telegram channel
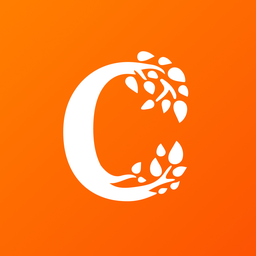
Full access? Get Clinical Tree
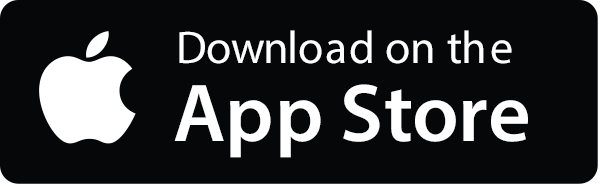
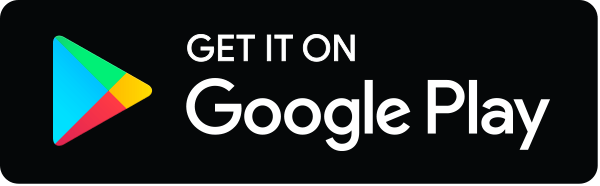