Abstract
Respiratory support for the child with critical heart disease remains one of the most important aspects of postoperative care. As devices have changed, so have the recommendations on how to use these new choices. The clinician will need a strong knowledge of what options are available and when specific strategies are employed. As important as initiating and maintaining appropriate ventilation, weaning techniques have also changed and should be an important part of all management strategies. This chapter defines these strategies.
Key Words:
Graphics, high-frequency ventilation, oxygenation, ventilation, weaning
Respiratory Support
Classification of Positive Pressure Ventilators
A mechanical ventilator is designed to replace or support lung function by altering, transmitting, and directly applying energy in a predetermined way to perform the work of the thorax and lungs. Nomenclature to classify the essential features of positive pressure ventilators has been described by many authors and continues to be an area of confusion for practitioners not closely involved in the design and development of ventilators.
A classification system provides a common knowledge base of terms and concepts to facilitate the understanding, interpretation, and assessment of ventilator operating systems and performance characteristics. The classification system must be clinically relevant and accurately reflect the pattern of respiratory support a patient receives. This section will present a classification system as it supports the clinical practice of ventilatory care.
Power Input/Transmission.
Ventilator power input is either electrical or pneumatic (compressed gas). The transmission of input power is a function of the drive and control mechanisms of the ventilator. Ventilator classification focuses on the control variables, output parameters, and alarm systems as applied to their clinical utility. More specifically, clinicians focus on how each tidal volume (VT) is delivered to describe the type of respiratory support a patient receives.
Control Schemes and Control Variables.
Ventilator control variables address the physical qualities adjusted, measured, and/or used to manipulate the various phases of the ventilatory cycle. The four general control variables are inspiratory flow pattern, limit, trigger, and cycle. Classifying ventilators with this system may seem cumbersome and challenging to practitioners; however, this system is designed to address advances in ventilator operation, is based on the physiology of the ventilatory strategies, and provides a platform for new technology without confounding terminology.
Typically the control variables remain constant despite changes in ventilatory load. Therefore the ventilator sacrifices other preset variables (i.e., dependent variables) to keep the control variables constant despite changes in the patient’s compliance and resistance. The dependent variables depend on the controlled variable(s) as well as changes in compliance and resistance of the patient’s respiratory system. Although each manufacturer develops and refines its control scheme for the manipulation of these variables, commonalities exist between ventilators, which allow clinicians to describe the resultant respiratory patterns with common terminology.
Inspiratory Flow.
The inspiratory flow pattern sets the characteristics of gas flow during a positive pressure breath and affects the distribution of that breath within the patient’s respiratory system. Clinicians must consider several factors when selecting an inspiratory flow pattern for an individual patient. Airway pressures are dependent on the mechanical properties of the lungs and movement of gas into the lungs. The airway pressures generated during inspiration increase as flow enters the respiratory system and encounters the resistance of the airways. The gas volume must also overcome the elastic recoil of the lung. Therefore peak pressure = (flow)(R aw ) + (VT)(elastance), where R aw is the airway resistance and VT is the tidal volume. The shape of the inspiratory flow pattern as it delivers the positive pressure breath determines the shape of the pressure curve and the peak pressure generated. This can be predicted given knowledge of the characteristics of the various flow patterns and the pneumatic characteristics of the patient’s lungs, as subsequently described. As derived from the equation, the selection of an appropriate inspiratory flow pattern based on a patient’s pulmonary pathophysiology will improve the effectiveness of ventilation, reduce peak inspiratory pressure, and optimize mean airway pressure, while promoting patient-ventilator synchrony.
Fig. 23.1 illustrates typical inspiratory flow patterns available with positive pressure ventilators. Four inspiratory flow patterns exist, although only the first two are generally available on current ventilators. A square-wave pattern is produced by a constant flow of gas throughout inspiration. Variable decelerating flow is a waveform characterized by peak flow early in inspiration and then a decrease in flow until end-inspiration. This decrease in flow is generally curvilinear but may vary based on the specific programming of the ventilator. Ascending, accelerated flow patterns produce a ramp pattern with low flow at beginning inspiration and a linear increase in flow throughout inspiration with peak flow delivered at end-inspiration. A sine wave pattern is generated by a variable flow with a rapid increase during the early phase of inspiration, a peak at midinspiration, then a decrease in flow until end-inspiration.

The functional performance of the various inspiratory flow patterns remains constant across manufacturers but may be produced by dramatically different control schema. The flow pattern characteristics of a specific mechanical ventilator significantly influence the airway pressures generated. Clinicians should monitor inspiratory/expiratory (I:E) ratios when selecting flow patterns during volume-limited ventilation and adjust the peak flow to maintain an appropriate inspiratory time.
Gas flow always follows the path of least resistance. Alterations in inspiratory flow pattern affect the distribution of gas flow based on the underlying pathophysiology and anatomic considerations of the respiratory system. Ascending (accelerating) flow patterns deliver the highest flow at end-inspiration when the effects of resistance and elastance are increased. Ascending flow patterns produce higher peak pressures compared to other flow patterns and are generally no longer in clinical use. A decelerating flow pattern has several advantages over an ascending pattern. Decelerating flow patterns deliver the highest flow at the beginning of inspiration when volume and elastance are low. Inspiratory flow then decreases during inspiration as delivered volume increases. Therefore peak airway pressure is lower, but mean airway pressure is higher. In general, as the maximum flow moves from the beginning to the end of the inspiratory cycle, peak pressure increases and mean airway pressure decreases.
Inspiratory flow patterns should be matched to each patient’s clinical condition. In situations in which the patient has high airway resistance (e.g., asthma, bronchiolitis, large airway obstruction), peak airway pressures may be reduced by avoiding a flow pattern with high peak inspiratory flow rates. In these patients a square-wave, constant flow pattern may generate a lower peak pressure than a descending flow pattern as a result of the decrease in peak flow. The actual effects in individual patients may vary widely with those patients who have a strong inspiratory demand being more comfortable with the generally high flow rate of a variable, decelerating pattern. In contrast, respiratory pathology characterized by a low compliance may benefit from a descending flow pattern in which the peak pressure is reduced but the mean airway pressure is increased. Variable flow ventilation (e.g., pressure control ventilation [PCV]) uses a decelerating flow pattern, which can significantly decrease the peak inspiratory pressure and increase the mean airway pressure to recruit collapsed alveoli and potentially improve oxygenation.
Trigger.
The inspiratory phase of a breath can be initiated by (1) patient effort, as determined by a change in either pressure or flow in the ventilator circuit, or (2) time. Therefore mechanical breaths may be pressure, flow, or time triggered. Flow triggering increases the sensitivity of the ventilator to the patient’s spontaneous demands and can decrease the response time of VT delivery. The ventilator “sensitivity” determines the degree of inspiratory effort a patient must exert to trigger the ventilator to deliver a VT. Ideally, this should be adjusted to 0.1 to 3.0 L/min of flow or −0.5 to −1.5 cm H 2 O to minimize the patient’s imposed work of breathing.
Cycle.
The cycle variable determines when inspiration ends. This variable is used as a feedback signal to terminate gas flow and allow the patient to passively exhale. Time is the most common cycle variable for mechanical breaths (time cycled). Certain spontaneous breaths (e.g., pressure supported breaths) can be flow cycled. In a flow-cycled breath the expiratory valve opens when inspiratory flow decreases to a preset percentage of peak inspiratory flow. This algorithm is generally preset, not adjustable, and varies among ventilators.
Limit.
During inspiration, pressure, volume, and flow increase above the end-expiratory values. Limit variables allow the clinician to control the upper limits of pressure and/or volume of the mechanical breath a patient receives, hence the description “pressure limited” and “volume limited.”
Ventilatory Modes
Using the terminology described earlier, a system can be developed that describes the ventilatory modes and specific breathing patterns used during positive pressure ventilation (PPV). Ventilatory modes may include mechanical breaths, spontaneous breaths, or a combination of both.
Mechanical Breaths.
Assist control mode delivers a patient-triggered ventilator VT with each spontaneous effort. In an assist control mode the ventilatory rate is determined by the patient and therefore may be in excess of the preset control rate. When there is no spontaneous respiratory effort, the minimum ventilatory rate is that which is set by the clinician. Assist control modes should be contrasted with support modes of ventilation. In both modes a predetermined level of support is adjusted by the clinician. In assisted modes the inspiratory time is determined by the clinician. In contrast, in supported modes the inspiratory time is determined by the patient. Assist control breaths may be limited by either volume or pressure. A patient may receive volume control ventilation (VCV), in which a predetermined minimum breath rate is delivered and breaths are volume limited, or PCV, in which a preset minimum breath rate is delivered along with a preset pressure limit.
In previous modes of ventilation the decelerating flow pattern was available only on the PCV mode. Now several vendors have combined the attributes of a decelerating flow pattern with a volume guarantee. In these modes, the ventilator guarantees a preset minute ventilation. In one such mode, pressure-regulated volume control (PRVC), the ventilator monitors the airway resistance and compliance of the lungs and adjusts the inspiratory pressure level via a predetermined algorithm to deliver a preset volume limit. PRVC provides the benefit of a stable minute ventilation while providing the opportunity to use a decelerating inspiratory flow pattern.
Spontaneous Breaths.
Spontaneous breathing may be supported with continuous positive airway pressure (CPAP), a mode in which the ventilator maintains a constant airway pressure throughout inspiration and expiration. A preset expiratory pressure limit prevents the patient from exhaling down to atmospheric pressure at end-expiration. Continuous or demand flow during inspiration maintains airway pressure above atmospheric pressure. Raising the expiratory pressure above atmospheric pressure increases end-expiratory lung volume (EELV) proportionate to the pressure applied and total respiratory compliance. The CPAP mode can be used alone or in conjunction with mechanical breaths. Of note, when used in combination with mechanical breaths, the term positive end-expiratory pressure (PEEP) is used instead of CPAP.
Pressure support ventilation (PSV) is a spontaneous breathing mode that can be used alone or in combination with other modes (synchronized intermittent mandatory ventilation [SIMV], CPAP). A preset level of inspiratory pressure is delivered above the baseline end-expiratory pressure with each spontaneous respiratory effort. PSV is initiated when pressure or flow decreases to the preset threshold level during inspiration. When this trigger is sensed by the ventilator, flow accelerates into the breathing circuit and increases proximal airway pressure to the preset pressure level. The pressure support breath is usually terminated when flow decreases to 25% of peak flow. The VT delivered in this mode will vary with changes in lung compliance, airway resistance, pressure support level, and inspiratory time.
Volume support (VS) combines the benefits of PSV with a volume guarantee during spontaneous breathing. The ventilator monitors airway resistance and pulmonary compliance and adjusts the pressure support level using a predetermined algorithm to deliver a preset minute ventilation. In this mode the pressure used to deliver the VT is automatically adjusted (a dependent variable).
Combined Breaths.
SIMV modes combine mechanical breaths with spontaneous breaths. Flow for spontaneous breathing may be provided by a continuous flow of gas through the breathing circuit, a demand valve, or a combination of both. If PEEP is applied with continuous and demand flow, the spontaneous breaths become CPAP breaths. Pressure support is often administered in conjunction with SIMV.
Neonatal and Pediatric Ventilators
In this classification system the mechanical breaths produced by most traditional neonatal ventilators would be considered constant flow, time-triggered, time-cycled, and pressure-limited breaths. The advances in neonatal ventilator design have enabled most modes that are available on pediatric and adult ventilators. Improvements in calibration and the measurement of smaller volumes have facilitated volume-limited mechanical SIMV breaths with CPAP or pressure support. Mechanical breaths can be pressure or flow triggered, time cycled, and volume or pressure limited. Spontaneous breaths are constant flow, CPAP, or variable flow, pressure-supported breaths.
The primary difference between neonatal and pediatric/adult ventilators is the range of flows and volumes the ventilator can deliver. Neonatal ventilators are able to deliver lower flows and volumes at faster rates and deliver breaths with a shorter response time to patient-triggered effort. Pediatric ventilators are essentially the same ventilators used in adults, only at lower ranges of flow and volume.
As technology has advanced, ventilator manufacturers have developed ventilators that are capable of ventilating patients ranging from small neonates to large adults. Table 23.1 contains the common classifications of mechanical ventilator breaths by mode of ventilation.
Mode | Mechanical Breath Variables | Spontaneous Breath Variables | ||||
---|---|---|---|---|---|---|
Trigger | Cycle | Limit | Trigger | Cycle | Limit | |
Control ventilation | Time | Time | Volume | — | — | — |
Flow | Pressure | |||||
Pressure | ||||||
Synchronized intermittent mandatory ventilation | Time | Time | Volume | Flow | Flow | Pressure |
Flow | Pressure | Pressure | ||||
Pressure | ||||||
Supported ventilation (PSV or VS) | — | — | — | Flow | Flow | Pressure |
Pressure | Volume |
Output Waveform Analysis
Ventilators provide continuous monitoring of respiratory mechanics, including displays of gas flow, volume delivery, and airway pressure. Output waveforms are a useful tool for understanding the characteristics of ventilator operation and provide a graphic display of the various modes of ventilation. Waveform analysis can be used to optimize mechanical ventilatory support and analyze ventilator incidents and alarm conditions. Using this technology, it is possible to tailor the form of ventilatory support, improve patient-ventilator synchrony, reduce patient work of breathing, and calculate a variety of physiologic parameters related to respiratory mechanics.
The most useful waveforms are flow, pressure, and volume graphed over time (termed scalars ). Convention dictates that positive values correspond to inspiration and negative values to expiration; horizontal axes represent time in seconds, and vertical axes represent the measured variable in its common unit of measurement. Optimal measurements are obtained when the pressure and flow monitoring device is positioned between the endotracheal tube (ETT) and the ventilator circuit. Measurements may also be obtained from the inspiratory or expiratory limbs of the ventilatory circuit. Integration of intrapleural pressure from an esophageal balloon further enhances graphic data and enables assessment of the patient’s work of breathing. During spontaneous breathing, patient effort, work of breathing, and the level of intrinsic PEEP are best evaluated using esophageal pressure measurements.
In addition to plotting flow, pressure, and volume versus time, each of these parameters can be plotted against each other. Pressure-volume and flow-volume loops can be particularly helpful in assessing alterations in resistance or compliance, work of breathing, overdistention of the lung, and intrinsic PEEP.
Flow Graphics.
Flow sensors should be capable of measuring a wide range of flows (−300 to +150 L/min) and be resistant to motion artifact, moisture, and respiratory secretions. The flow graphic has two distinct parts: inspiratory flow and expiratory flow. The inspiratory flow graphic displays the magnitude, duration, and flow pattern of the positive pressure breath or spontaneous breath. Fig. 23.2 is a theoretical inspiratory flow pattern of a continuous flow mechanical breath. In actual application, flow delivery mechanisms have response times that alter the shape of the flow graphic. These response times result in a positive slope at the start of inspiration and a negative slope at end-inspiration ( Fig. 23.3 ).


The flow graphic of a spontaneous breath is demonstrated in Fig. 23.4 . The characteristic of the flow graphic is determined by the characteristics of the patient’s inspiratory demand and the ventilatory support provided to the spontaneous breath (i.e., continuous flow CPAP, demand flow CPAP, and/or pressure support).

The expiratory flow of gas is generally passive for mechanical and spontaneous breaths, although patients may forcibly exhale to assist exhalation. The magnitude, duration, and pattern of the expiratory graphic are determined by the resistance of the patient’s respiratory system and ventilator circuit. Important features of the ventilator circuit that affect the flow graphic include the size and length of the ETT, internal diameter and length of the ventilator circuit, resistance of the expiratory valve, and distensibility of the circuit itself. Fig. 23.5 represents a typical expiratory flow graphic for a positive pressure breath. The expiratory flow, by convention, is shown below the zero baseline. Because the characteristics of the patient circuit that affect the expiratory flow pattern are generally fixed, dramatic changes in the expiratory flow curve may be attributable to changes in the patient’s resistance, compliance, or activity. For example, an increase in airway resistance due to obstructive disease or secretions may result in decreased peak expiratory flow, increased duration of flow, or failure of flow to return to baseline ( Fig. 23.6 ).


Pressure Graphics.
Although resistance of the ETT is a component of the pressure graphic, pressures measured are generally considered to reflect airway pressure. In a typical pressure-triggered breath from a demand-flow valve, there is a slight pressure drop at the beginning of inspiration, and the magnitude of the drop is proportionate to the patient’s peak inspiratory flow rate, sensitivity of the demand valve, and response of the flow delivery system. Of note, this pressure drop usually is not seen in a flow-triggered breath. During a mechanically supported breath the peak inspiratory pressure is determined by the patient and circuit compliance, resistance, delivered VT, and inspiratory flow ( Fig. 23.7 ). Baseline pressure reflects the expiratory pressure in the circuit (i.e., PEEP or CPAP). The pressure-time graphic is useful for evaluating the stability of PEEP in the presence of an air leak.

Volume Graphics.
Volume is generally measured by integrating the flow signal with inspiratory time ( Fig. 23.8 ). The upsweep of the graphic represents the volume delivered to the patient and/or circuit. The “downsweep” of the graphic represents the total expiratory volume. Typically, inspiratory and expiratory volumes should be equal. Nevertheless, it is not uncommon in infants and children with uncuffed ETTs for the expiratory volume to be less than the inspiratory volume. An actual percentage leak can be calculated and may aid in the decision to change the ETT size.

Patient-Ventilator Synchrony.
The timing sequence of various respiratory events can be determined by displaying volume, flow, and pressure over time ( Figs. 23.9 and 23.10 ). Comparisons of all three graphics simultaneously facilitate analysis of ventilator-patient interactions. Ventilator dyssynchrony becomes evident when the timing and magnitude of flow, pressure, and volume are disproportionate or delayed.




Ventilator Parameters and Alarm Systems
Parameters.
Spontaneous VT and respiratory rate are a reflection of circuit characteristics, respiratory system compliance and resistance, and respiratory muscle function. Assessment of mechanical VT and preset rate ensures delivery of the prescribed alveolar ventilation and facilitates detection of endotracheal or ventilator circuit leaks. Inspiratory time is selected by the clinician to facilitate patient comfort and synchronous breathing during PPV. The patient’s age and respiratory pattern are major considerations in the selection of inspiratory time. Recommended inspiratory times by age-group are as follows:
Newborns: 0.3 to 0.5 seconds
Toddlers: 0.5 to 0.75 seconds
Children: 0.75 to 1.0 seconds
Adults: 0.75 to 1.5 seconds
Total cycle time is the time allotted for one complete inspiratory (I) and expiratory (E) cycle. I:E ratio is an expression of the set inspiratory time and the remaining expiratory cycle time. Recommended I:E ratios vary greatly with ventilator rate. Ratios of 1 : 2 or 1 : 3 are most desirable but should be no lower than 1 : 1 in assisted ventilation to allow adequate time for exhalation. Peak flow should be titrated to the spontaneous demands of the patient.
Peak inspiratory pressures during volume-limited ventilation are a reflection of volume, flow, inspiratory time, airway resistance, and respiratory system compliance. Peak inspiratory pressures vary with alterations in the patient’s respiratory physiology. The presence of airway secretions, bronchospasm, tubing kinks, pneumothorax, agitation, and decreased lung compliance all may increase peak inspiratory pressures. Decreased peak pressures reflect an air leak around the ETT, a leak in the ventilator circuit, or an improvement in the child’s lung mechanics. Peak pressure is not an indicator of changes in patient condition with time-cycled, pressure-limited ventilation.
Mean airway pressure closely reflects alveolar pressure and is an important indicator of the degree of PPV required to achieve adequate oxygenation. Mean airway pressure is principally a function of inspiratory time and PEEP; however, VT, peak inspiratory pressure, inspiratory flow pattern, and ventilator rate also play a role.
Plateau pressure is obtained by recording the pressure following an inspiratory hold maneuver. Plateau pressure reflects the compliance of the lung without gas flow and eliminates the airway resistance component. A plateau pressure must be obtained with a constant inspiratory flow pattern and cannot be measured in the presence of an air leak.
PEEP is produced by closure of the ventilator expiratory valve. The volume of gas remaining in the lung is proportionate to the end-expiratory pressure and the patient’s compliance. Increasing the volume of gas increases EELV.
Alarms.
Ventilator alarm systems have improved dramatically with microprocessor technology. Input power alarms notify clinicians of changes in electrical or pneumatic supplies. Control circuit alarms notify the clinician of incompatible parameters or that the ventilator self-test has failed. Output alarms indicate unacceptable levels of ventilator output, including peak airway pressure, end-expiratory pressure, volume, flow, minute ventilation, respiratory rate, and inspired gas concentration. The fraction of inspired oxygen (FiO 2 ) should be analyzed continuously with high and low alarm limits set to prevent inadvertent hyperoxemia or hypoxemia.
Nonconventional Modes of Ventilation
High-Frequency Ventilation.
High-frequency ventilation (HFV) refers to a variety of technologies that use VTs smaller than the patient’s anatomic dead space and high ventilatory frequencies to minimize the effects of increased peak pressure. HFV consists of a variety of ventilatory strategies, including high-frequency oscillatory ventilation (HFOV) and high-frequency jet ventilation (HFJV).
High-Frequency Oscillatory Ventilation.
HFOV uses an electrically powered piston diaphragm oscillator to alternate positive and negative pressures in the airway. With use of this diaphragm, VTs between 1 and 3 mL/kg are generated with cycles ranging from 300 to 900 beats/min (5 to 15 Hz). During HFOV, inspiration and expiration are both active and occur above and below the mean airway pressure baseline. HFOV has been used successfully in neonates with respiratory distress syndrome and children with acute respiratory distress syndrome (ARDS). HFOV has also been used successfully in air leak syndrome in both neonates and children. Recently in a large pediatric observational study, HFOV was shown to increase length of ventilation and possibly increase mortality. The HFOV should be used with caution because the data have not shown improvements in outcomes.
The exact mechanisms of gas exchange during HFOV remain controversial. The potential mechanisms include convective gas transport, coaxial flow, Taylor dispersion, molecular diffusion, and the pendelluft effect. Conventional bulk flow is responsible for gas delivery to the larger airways and potentially to the alveoli close to these larger airways. Coaxial flow is the bidirectional flow of gas in the airways at the same time. A net flow of gas can occur in one direction through the center of the airway and in the other direction in the area closer to the airway wall. Taylor dispersion describes gas flow along the front of a high-velocity gas flow. Gas transport occurs as a result of gas dispersion beyond the bulk flow front. Molecular diffusion is known to occur at the alveolar level during conventional ventilation, and enhanced diffusion may play a role during HFV. The pendelluft effect is the phenomenon of intraunit gas mixing due to the impedance difference among lung units.
Any strategy that results in increased mean airway pressure may result in rapid transmission of the increased intrathoracic pressure to the cardiovascular structures and subsequently to cardiovascular compromise. HFOV should be used with caution in patients with congenital heart disease (CHD) associated with passive pulmonary blood flow and/or right ventricular (RV) dysfunction and in those with hemodynamic instability because these patients may be sensitive to the resulting higher mean airway pressures. Also, HFOV should be used with caution in those with severe asthma given the less efficient active expiratory flow.
High-Frequency Jet Ventilation.
Most HFJV systems use a high-pressure, air-oxygen gas source that generates gas flow. A rapid solenoid valve allows for flow interruption, which regulates the frequency of ventilation. A reducing valve is present that allows adjustments of inspiratory driving pressure from 10 to 50 psi. The current US Food and Drug Administration–approved HFJV system (Bunnell LifePulse Ventilator, Bunnell Inc., Salt Lake City, UT) requires a second ventilator in tandem.
During HFJV, peak inspiratory pressures can be controlled from 8 to 50 cm H 2 O, inspiratory time from 20 to 40 milliseconds, and respiratory rate from 150 to 600 insufflations per minute. The PEEP and sigh breaths are regulated by the tandem ventilator. Previously a specific ETT was necessary for HFJV, which required reintubation. This is no longer required because specific jet adapters are now used.
Effect on Gas Exchange.
Although the mechanism of gas exchange during HFJV has not been well defined, convection streaming and enhanced molecular diffusion are known to occur. Simply defined, convection streaming is the flow of gas in a bulk flow manner to the level of the alveoli. Gas is injected into the airway at high speed during HFJV. The gas molecules located in the center of the inspired gas travel faster than those at the edges (asymmetric velocity profiles). Exhalation is passive and promoted by an extremely short inhalation time (20 to 40 milliseconds) and long exhalation times. The exhaled gas travels at a slower velocity than the inspired gas. When exhaled gas encounters the rapidly moving inspired gas, it is extruded along the tracheal walls. The net result is continuous exhalation of gas around the inspired gas. Molecular diffusion is the rapid kinetic motion of molecules and occurs in the terminal bronchioles and alveoli. A variety of other theories have been proposed to explain the ability of HFJV to provide adequate ventilation at VTs below dead space.
Carbon Dioxide Elimination.
During PPV, manipulations in minute ventilation, ventilatory rate, and VT allow alterations in CO 2 elimination. During HFJV, CO 2 elimination is governed by the relationship (VT) a × f b , where VT = tidal volume, f = frequency. In this relationship a ranges from 1.5 to 2.5 and b from 0.5 to 1.0. Because a > b, alveolar ventilation during HFJV has a greater dependency on alterations in VT than frequency.
The primary method of eliminating CO 2 during HFJV is by increasing the delivered VT. Increasing the delivered VT can be accomplished by increasing the inspiratory pressures during HFJV. This increases alveolar ventilation and may improve ventilation/perfusion matching. If atelectasis develops, increasing the PEEP (i.e., mean airway pressure) may help recruit lung volume. PEEP is adjusted during HFJV on the tandem ventilator. The tandem ventilator is usually set to administer 0 to 10 sigh breaths per minute. The sigh breath should be set at a peak pressure less than that set on the HFJV (HFJV breaths are not interrupted) and should not exceed 30 cm H 2 O. In patients, sigh breaths are designed to prevent atelectasis and allow for appropriate recruitment of lung volume. Alternatively, EELV may be maintained without any sigh breaths if the PEEP (i.e., mean airway pressure) is titrated upward. When the PaCO 2 becomes elevated, increasing the HFJV peak pressure (i.e., VT) may be necessary to increase alveolar ventilation.
During HFJV, VT is dependent on the respiratory frequency, which also affects CO 2 elimination. When the respiratory frequency is increased, a reduction of VT may occur, resulting in decreased tidal alveolar ventilation. Therefore under certain conditions, increasing the respiratory frequency may result in a reduction in alveolar ventilation. For these reasons, manipulations of VT remain the most important determinant of alveolar ventilation during HFJV.
In premature infants, HFJV is initiated at frequencies of 360 to 480 insufflations per minute. This can be accomplished because the premature lung has a short time constant, and b approximates 1.0. In premature infants, increasing the respiratory frequency results in improved alveolar ventilation. In older patients and those with compliant lungs the lung has a longer time constant, and b approaches 0.5. In comparison with premature patients, increasing the respiratory frequency in older patients and patients with normal compliance results in a reduction of the delivered VT and an overall reduction of alveolar ventilation. For these reasons HFJV is usually begun at a frequency of 240 to 300 insufflations per minute in children with respiratory or cardiovascular dysfunction.
Lung Volumes.
During HFJV, lung volumes do not vary dramatically because peak pressures are low, inspiratory time is short, and mean airway pressure is constant. Mean lung volume is determined by the mean airway pressure. Therefore lung volumes remain relatively static around the mean lung volume. During HFJV, oxygenation is primarily dependent upon mean airway pressures, and increasing mean airway pressures will increase lung volume and improve ventilation/perfusion (V̇/Q̇) matching. Mean airway pressure is most affected by increasing the PEEP on the tandem ventilator. Peak inspiratory pressure of the jet ventilator and the VT and rate of the sigh breaths play a lesser role.
Effect on Cardiovascular Parameters.
The primary physiologic effect of HFJV is improved ventilation at equivalent mean airway pressures developed during conventional mechanical ventilation. Therefore during HFJV the mean airway pressures can be reduced while maintaining alveolar ventilation and CO 2 clearance, thus limiting the potential adverse effects of positive intrathoracic pressure on cardiovascular performance.
Negative Pressure Ventilation.
Negative pressure ventilation (NPV) can be used in patients after surgery for CHD and in pediatric patients with respiratory dysfunction. Currently NPV is usually performed using a chest cuirass that covers the patient’s chest and abdomen. Negative pressure is generated within this cuirass. Such cuirass devices avoid the limitations of body tank devices (iron lung type of devices), which are rarely used today. The advantage of NPV is that intubation is avoided, sedation requirements may be decreased, and systemic venous return may be improved. However, the disadvantage of NPV is that left ventricular (LV) afterload may be increased. The regulation of respiratory parameters during NPV, including I:E ratios, can be difficult. NPV is not routinely used in patients with CHD; however, it may be an effective technique in patients with passive pulmonary blood flow such as following Glenn shunt and Fontan procedures.
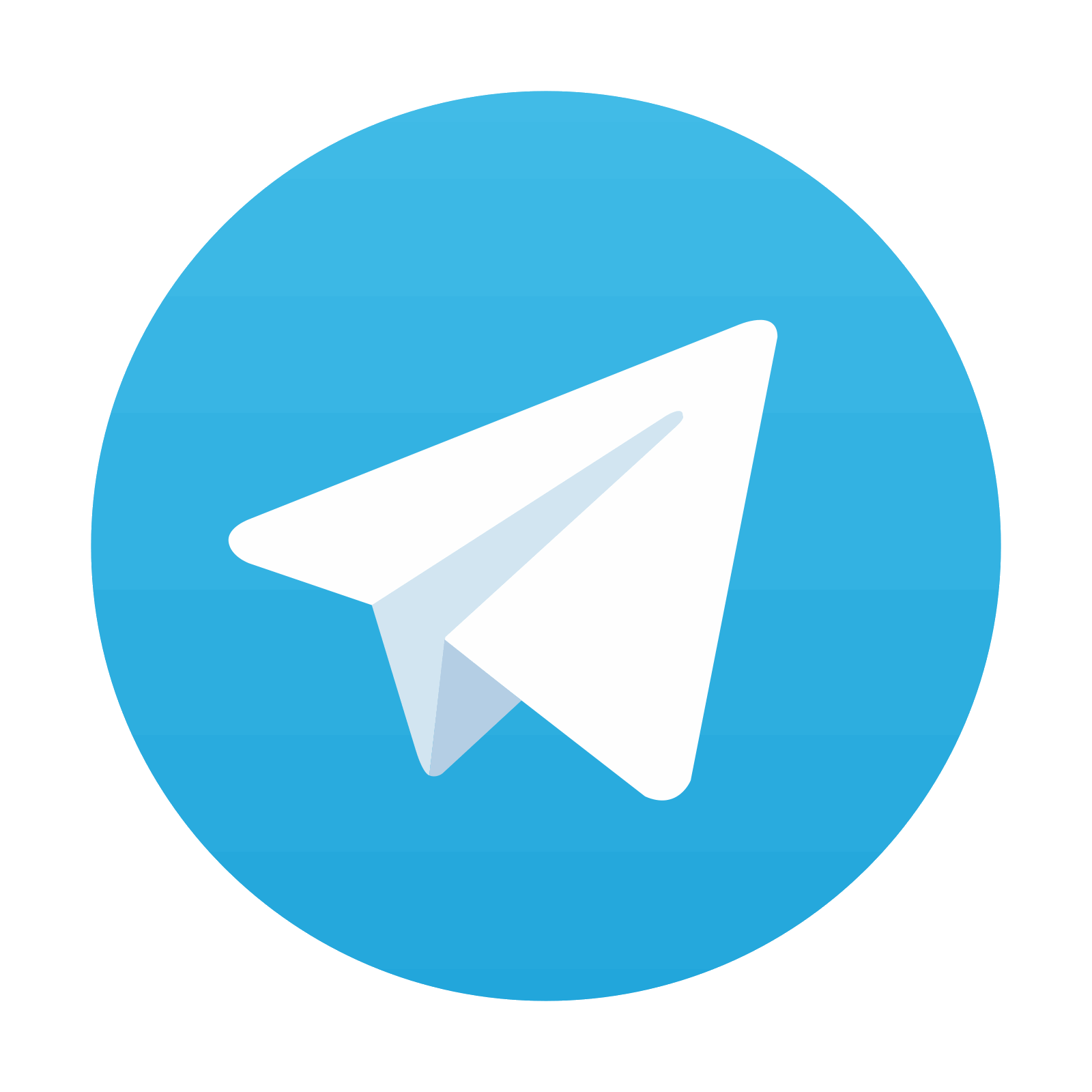
Stay updated, free articles. Join our Telegram channel
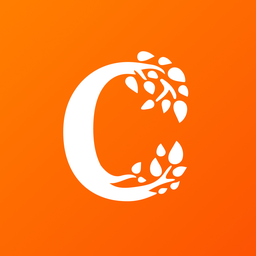
Full access? Get Clinical Tree
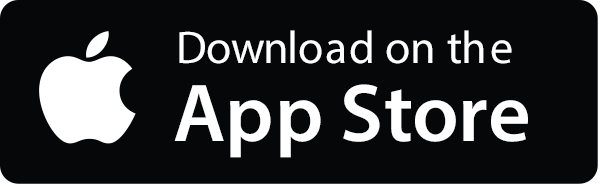
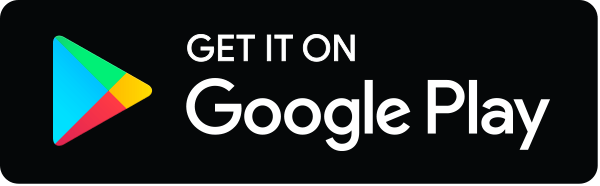