11
Valvular Stenosis
Diagnostic Imaging of the Aortic Valve
Quantitation of Aortic Stenosis Severity
Response of the Left Ventricle
Decisions About Timing of Intervention
Disease Progression and Prognosis in Asymptomatic Aortic Stenosis
Evaluation of Aortic Stenosis with Left Ventricular Systolic Dysfunction
Diagnostic Imaging of the Mitral Valve
Quantitation of Mitral Stenosis Severity
Consequences of Mitral Stenosis
Clinical Applications in Specific Patient Populations
Basic Principles
Approach to the Evaluation of Valvular Stenosis
Complete echocardiographic evaluation of the patient with valvular stenosis includes:
Fluid Dynamics of Valvular Stenosis
The fluid dynamics of a stenotic valve are characterized by the formation of a laminar, high-velocity jet in the narrowed orifice. The flow profile in cross section at the origin of the jet is relatively blunt (or flat) and remains blunt as the jet reaches its narrowest cross-sectional area in the vena contracta, slightly downstream from the anatomic orifice (Fig. 11-1). Thus the narrowest cross-sectional area of flow (physiologic orifice area) is smaller than the anatomic orifice area. The magnitude of the difference between physiologic and anatomic area depends on orifice geometry and the Reynolds number (a descriptor of the inertial and shear stress properties of the fluid). The ratio of the physiologic to anatomic orifice area is known as the discharge coefficient.
Figure 11–1 Fluid dynamics of the stenotic aortic valve.
The LV outflow tract is bounded by the septum and anterior mitral valve leaflet (AMVL). As LVOT flow accelerates and converges, a relatively flat velocity profile occurs proximal to the stenotic valve, as indicated by the arrowheads. Flow accelerates in a spatially small zone adjacent to the valve as blood enters the narrowed orifice. In the stenotic orifice, a high-velocity laminar jet is formed with the narrowest flow stream (vena contracta, indicated by the blue line) occurring downstream from the orifice. Beyond the jet, flow is disturbed, with blood cells moving in multiple directions and velocities. (Reprinted with permission from Judge KW, Otto CM: Doppler echocardiographic evaluation of aortic stenosis. Cardiol Clin 8:203, 1990.)
The length of the high-velocity jet also is dependent on orifice geometry and can be variable in the clinical setting with, for example, a very short jet across a deformed, irregular, calcified aortic valve and a longer jet across a smoothly tapering, symmetric, rheumatic mitral valve or a congenitally stenotic semilunar valve (Fig. 11-2).
Figure 11–2 Mitral stenosis (MS) jet on color flow imaging.
The apical four-chamber view shows a long jet directed toward the LV apex with a proximal isovelocity surface area on the LA side of the valve.
Relationship Between Pressure Gradient and Velocity
where ΔP is the pressure gradient across the stenosis (mm Hg), ρ is the mass density of blood (1.06 × 103kg/m3), v2 is velocity in the stenotic jet, v1 is the velocity proximal to the stenosis, (dv/dt)dx is the time-varying velocity at each distance along the flowstream, and R is a constant describing the viscous losses for that fluid and orifice. Historically, Daniel Bernoulli first described this equation in 1738 from studies of steady water flow in rigid tubes. The concepts were later expanded and refined by Euler. Of note, these equations may not be strictly applicable to pulsatile blood flow in compliant chambers and vessels, although clinical studies have shown that remarkably accurate pressure gradient predictions can be made with this approach. This equation was first applied to Doppler data by Holen in 1976 for stenotic mitral valves and by Hatle in 1979 for stenotic aortic valves.
Distal Flow Disturbance
Distal to the stenotic jet, the flowstream becomes disorganized with multiple blood flow velocities and directions, although fully developed turbulence, as strictly defined in fluid dynamic terms, may not occur. The distance that this flow disturbance propagates downstream is related to stenosis severity. In addition, the presence of a downstream flow disturbance can be extremely useful in defining the exact anatomic site of obstruction, for example, allowing differentiation of subvalvular outflow obstruction (flow disturbance on the ventricular side of the valve) from valvular obstruction (flow disturbance only distal to the valve) (Fig. 11-3).
Figure 11–3 Level of outflow obstruction.
Color flow imaging in calcific valvular aortic stenosis in a parasternal long-axis view (left) with the poststenotic flow disturbance identifying the site of obstruction at the valvular level. In contrast, in a patient with subaortic stenosis (right), flow acceleration occurs proximal to the valve. A membrane is not seen on these images but was demonstrated on TEE. Ao, aorta.
Proximal Flow Patterns
In contrast, the flow pattern proximal to the stenotic mitral valve is quite different (Fig. 11-4). Here, the left atrial (LA) to LV pressure gradient drives flow passively from the large inlet chamber (the LA) abruptly across the stenotic orifice. Proximal flow acceleration is prominent over a large region of the LA. The three-dimensional (3D) velocity profile is curved; that is, flow velocities are faster adjacent to and in the center of a line continuous with the jet direction through the narrowed orifice and slower at increasing radial distances from the valve orifice. The proximal velocity profile of an atrioventricular valve thus is hemielliptical, unlike the more flattened velocity profile proximal to a stenotic semilunar valve. Any 3D surface area proximal to a narrowed orifice at which all the blood velocities are equal can be referred to as a proximal isovelocity surface area (PISA).
Figure 11–4 Fluid dynamics of rheumatic mitral stenosis.
The stream lines of flow accelerate as they approach the stenotic orifice, with several curved proximal isovelocity surface areas indicated. The mitral stenosis jet is long, with the postjet flow disturbance occurring adjacent and distal to the laminar jet.
The clinical importance of these flow patterns is that stroke volume can be calculated proximally to a stenotic valve based on knowledge of the cross-sectional area of flow and the spatial mean flow velocity over the period of flow, as described in Chapter 6. This concept applies to the flat flow profile proximal to a stenotic aortic valve (used in the continuity equation), to the proximal flow patterns seen in mitral stenosis, and to the proximal isovelocity surface areas seen with regurgitant lesions (see Chapter 12).
Aortic Stenosis
Diagnostic Imaging of the Aortic Valve
Aortic valve stenosis (Fig. 11-5) in adults most often is due to:
Figure 11–5 Causes of aortic stenosis.
In a parasternal mid-systolic short-axis view, calcific aortic stenosis is characterized by fibro-calcific masses on the aortic side of the leaflet that result in increased leaflet stiffness without commissural fusion. Calcific shadowing and reverberations limit image quality. With a congenital bicuspid valve, the two leaflets (with a raphe in the anterior leaflet) open widely in systole. The diagnostic features of rheumatic stenosis are commissural fusion and mitral valve involvement, with the characteristic triangular aortic valve opening in systole. The unicupsid valve has only one point of attachment (at the 6 o’clock position) with a funnel-shaped valve opening.
Calcific stenosis of a trileaflet or congenital bicuspid valve
Congenital valve disease (bicuspid or unicuspid)
Calcific Aortic Stenosis
About 25% of all adults over age 65 years have aortic valve “sclerosis”—areas of increased echogenicity, typically at the base of the valve leaflets, without significant obstruction to LV outflow. About 10% to 15% of these patients have progressive leaflet thickening over several years resulting in significant obstruction to LV outflow, typically presenting at 70 to 85 years of age. When obstruction is present, imaging shows a marked increase in echogenicity of the leaflets consistent with calcific disease and reduced systolic opening. Direct measurement of valve area on short-axis two-dimensional (2D) or 3D imaging is possible in some patients either with excellent transthoracic (TTE) images or from a transesophageal echocardiographic (TEE) approach. However, directly planimetered aortic valve areas should be interpreted with caution because of the complex anatomy of the orifice and calcific shadowing and reverberation, even with 3D imaging. It is critical to ensure that the narrowest orifice of the valve is visualized and nonplanar geometry is considered. Even when carefully performed, direct measurement of valve area on imaging reflects anatomic valve area, whereas Doppler data provide functional valve area (Fig. 11-6).
Figure 11–6 Measurement of aortic valve area by 3D transesophageal echocardiography.
The tip of the aortic valve was obtained as the smallest possible area (A1, A2). The shape and area of the aortic valve changed (from A1 to B1) as the green plane moved slightly from the tip to the base (from A2 to B2). Dotted lines indicate aortic valve area at each level. Ao, ascending aorta. (From Saitoh T, Shiota M, Izumo M, et al: Comparison of left ventricular outflow geometry and aortic valve area in patients with aortic stenosis by 2-dimensional versus 3-dimensional echocardiography. Am J Cardiol 109[11]:1626-1631, 2012.)
Bicuspid Aortic Valve
A congenital bicuspid valve accounts for two thirds of cases of severe aortic stenosis in adults younger than 70 and one third of cases in those over age 70 years. Secondary calcification of a bicuspid aortic valve can be difficult to distinguish from calcification of a trileaflet valve once stenosis becomes severe; however, earlier in the disease course, a bicuspid valve can be identified on 2D parasternal short-axis views by demonstrating that there are only two open leaflets in systole (Fig. 11-7). Long-axis views show systolic bowing of the leaflets into the aorta, resulting in a “domelike” appearance. M-mode recordings may help in identifying a bicuspid valve if an eccentric closure line is present but can be misleading in terms of the degree of leaflet separation if the M-mode recording is taken through the base, rather than the tips, of the bowed leaflets. Similarly, planimetry of valve area may be erroneous if the image plane is not aligned with the narrowest point at the leaflet tips. Three-dimensional imaging is helpful in the identification of bicuspid valve anatomy when the diagnosis is not clear.
Figure 11–7 Bicuspid aortic valve.
Diastolic (top) and systolic (bottom) frames in a parasternal long-axis (PLAX) view show diastolic sagging and systolic doming of the leaflets. In the parasternal short-axis (PSAX) view, only two leaflets (arrows) are seen to open in systole with the commissures at four o’clock and ten o’clock positions. Ao, aorta; RVOT, RV outflow tract.
The most common bicuspid valve phenotype (seen in 70% to 80% of patients) is a larger anterior leaflet with the valve opening along an anterolateral-posteromedial closure line due to congenital fusion of the right and left coronary cusps (Fig. 11-8). A larger rightward leaflet with the closure line running anterior-posterior due to congenital fusion of the right and noncoronary cusps accounts for about 20% to 30% of cases. Fusion of the noncoronary and left coronary cusps, with a medial-lateral closure line, is least common. Many bicuspid valves have a raphe in the larger leaflet, so the closed valve in diastole appears trileaflet; accurate identification of the number of aortic valve leaflets can be made only in systole. Doppler interrogation of the aortic valve should be performed whenever a bicuspid valve is suspected to evaluate for stenosis, regurgitation, or both. Bicuspid aortic valve disease often is associated with dilation of the aortic sinuses and ascending aorta, with the pattern and severity of aortic dilation related to valve morphology.
Figure 11–8 Bicuspid valve classification.
Schematic diagram of the different bicuspid aortic valve phenotypes drawn in an orientation similar to a TTE parasternal short-axis view. Positions of the right coronary artery (RCA) and left coronary artery (LCA) ostia are shown. The A-P phenotype shows anterior-posterior leaflet orientation with fusion of the right and left coronary cusps. The R-L phenotype shows right and left leaflet orientation with fusion of the right and noncoronary cusps. A raphe (dotted line) may or may not be present. (From Schaefer BM, Lewin MB, Stout KK, et al: Usefulness of bicuspid aortic valve phenotype to predict elastic properties of the ascending aorta. Am J Cardiol 99[5]:686-690, 2007.)
Differential Diagnosis
The differential diagnosis of LV outflow obstruction includes:
In a patient with a clinical diagnosis of valvular aortic stenosis, the echocardiographic study should demonstrate whether the obstruction is, in fact, valvular or if one of these other diagnoses accounts for the clinical presentation (Fig. 11-9).
Figure 11–9 Different types of LV outflow obstruction.
Examples of the shape of the CW Doppler velocity curve in valvular aortic stenosis, fixed subvalvular obstruction due to a subaortic membrane, and dynamic obstruction due to hypertrophic cardiomyopathy. Note that the CW curves for subvalvular and valvular aortic stenosis are similar, although coarse fluttering of the valve with subvalvular obstruction results in a “rough” appearance of the systolic velocity curve. These can be distinguished by 2D and color flow imaging. The shape of the curve with dynamic obstruction is distinctly different, with the velocity peaking in late systole.
A subaortic membrane should be suspected in young adults when the valve anatomy is not clearly stenotic, yet Doppler examination reveals a high transaortic pressure gradient. Because the membrane may be poorly depicted on a transthoracic study, TEE imaging should be considered when this diagnosis is suspected (see Fig. 17-1). The spatial orientation of the jet and the shape of the continuous-wave (CW) Doppler velocity curve are similar for fixed obstructions, whether subvalvular, supravalvular, or valvular, but careful pulsed Doppler or color flow imaging allows localization of the level of obstruction by detection of the poststenotic flow disturbance and site of increase in flow velocity.
In dynamic outflow obstruction, the timing and shape of the late-peaking CW Doppler velocity curve are distinctive. In addition, the degree of obstruction changes dramatically with provocative maneuvers, as detailed in Chapter 9. In the occasional patient with both subvalvular and valvular obstruction, high-pulse repetition frequency Doppler ultrasound can be helpful in defining the maximum velocities at each site of obstruction.
Quantitation of Aortic Stenosis Severity
The severity of valvular aortic stenosis can be determined accurately using equations derived from our understanding of the fluid dynamics of a stenotic valve. Standard evaluation of stenosis severity includes:
Maximum Aortic Jet Velocity
Accurate measurement of aortic velocity requires a parallel intercept angle between the direction of the jet and the ultrasound beam. With a parallel alignment, cosine θ equals 1 and thus can be ignored in the Doppler equation (see Chapter 1). However, any deviation from a parallel intercept angle results in an underestimation of jet velocity. Although intercept angles within 15° of parallel will result in an error in velocity of 5% or less, an intercept angle of 30° will result in a measured velocity of 4.3 m/s when the actual velocity is 5 m/s. Underestimation of velocity, which is squared in the Bernoulli equation, results in an even larger error in calculated pressure gradient.
Care is needed to correctly identify the origin of the high-velocity jet. Other high-velocity systolic jets (Table 11-1 and Fig. 11-10) may be mistaken for aortic stenosis if inadequate attention is paid to timing, shape, and associated diastolic flow curves. In some cases, 2D-“guided” CW Doppler may be helpful in the correct identification of the jet, followed by recording with a nonimaging transducer for optimal signal quality.
TABLE 11-1
Other High-Velocity Systolic Jets That May Be Mistaken for Aortic Stenosis
Subaortic obstruction (fixed or dynamic)
Mitral regurgitation
Tricuspid regurgitation
Ventricular septal defect
Pulmonic or branch pulmonary artery stenosis
Peripheral vascular stenosis (e.g., subclavian artery)
Figure 11–10 Correct identification of the aortic jet.
From an apical approach, three different high-velocity systolic jets directed away from the transducer were recorded in this patient with moderate aortic stenosis, mild mitral regurgitation, and severe pulmonary hypertension. These three flow signals can be differentiated based on timing, shape, and associated diastolic flow signals.
Pressure Gradients
Maximum transaortic pressure gradient (ΔPmax) can be calculated from the maximum aortic jet velocity (Vmax) using the simplified Bernoulli equation (Fig. 11-11):
Figure 11–11 Pulsed Doppler recording of LV outflow tract velocity (top) and CW Doppler recording of an aortic stenosis jet (bottom).
The CW Doppler was recorded from an apical approach with a dedicated nonimaging transducer after evaluation from several windows with careful angulation to identify the highest velocity jet. This represents the most parallel intercept angle between the direction of blood flow and the stenotic jet. The maximum pressure gradient is calculated as ΔP=4v2, with mean pressure gradient determined by integrating the instantaneous gradients over the systolic ejection period. The velocity ratio is 0.26.
With careful attention to technical details, Doppler-determined pressure gradients are accurate, as has been demonstrated in numerous in vitro and animal models and in clinical studies (Table 11-2). Although Doppler maximum gradients correspond to maximum instantaneous gradients by catheter measurement, and Doppler mean gradients correspond to catheter-measured mean gradients, neither Doppler gradient correlates with the peak-to-peak gradient reported at catheterization. In fact, peak aortic and peak LV pressures do not occur simultaneously, so none of the instantaneous velocities recorded with Doppler ultrasound are strictly comparable with this invasive measurement. Potential confusion about Doppler pressure gradient data in an individual patient can be avoided by only comparing mean gradients (Fig. 11-12).
TABLE 11-2
Data sources: Callahan et al: Am J Cardiol 56:989-993, 1985; Smith et al: J Am Coll Cardiol 6:1306-1314, 1985; Currie et al: Circulation 71:1162-1169, 1985; Smith et al: Am Heart J 111:245-252, 1986; Simpson et al: Br Heart J 53:636-639, 1985; Burwash et al: Am J Physiol 265 (Heart Circ Physiol 34):H734-H1743, 1993.
Figure 11–12 LV and aortic (Ao) pressures in aortic valve stenosis.
LV and aortic pressures were measured directly with fluid-filled catheters in a patient with severe valvular aortic stenosis. Note that the maximum instantaneous gradient is greater than the peak-to-peak gradient. Mean gradient is indicated by the shaded area.
Physiologic changes in pressure gradient should be taken into consideration when comparing nonsimultaneous data recordings and inpatient management decisions. Pressure gradients depend on volume flow rate in addition to the degree of valve narrowing, so in an individual patient the pressure gradient will rise when transaortic stroke volume increases (e.g., anxiety, exercise) and will fall when stroke volume decreases (e.g., sedation, hypovolemia).
Continuity Equation Valve Area
If flow is laminar with a spatially flat velocity profile,
where CSA is the cross-sectional area of flow (cm2), SV is stroke volume (cm3), and VTI is the velocity-time integral (cm). Because flow both proximal to and in the aortic jet itself is laminar with a reasonably flat velocity profile,
Thus, the measurements needed to calculate valve area with the continuity equation (Fig. 11-13) are as follows:
Figure 11–13 Continuity equation aortic valve area (AVA).
Calculation require measurement of LV outflow tract (LVOT) diameter from a parasternal long-axis view for circular cross-sectional area (CSA) calculation (above, right) and pulsed Doppler recording of the LV outflow tract velocity-time integral (VTI) from an apical approach (above, left) to calculate transaortic stroke volume (SV). The aortic stenosis jet (ASjet) signal is recorded with CW Doppler from whichever window gives the highest maximum velocity.
The simplified continuity equation, then, is:
Potential Pitfalls
Continuity equation valve areas have been well validated in comparison with Gorlin formula valve areas calculated from invasive measurements of pressure gradient and cardiac output (Table 11-3). Some of the discrepancies between Doppler echo and invasive measurements of valve area are due to measurement variability for the invasive data and to limitations of the Gorlin formula itself. However, technical considerations in recording the Doppler and 2D echo data and the measurement variability of the noninvasive technique also are important (Table 11-4). Each laboratory should confirm the accuracy of its data by comparison with those of an experienced echocardiography laboratory or with other diagnostic tests.
TABLE 11-3
Selected Studies of Aortic Valve Area Determination
∗If not stated in the publication, statistics were calculated from the raw data provided in tables. A blank indicates that data for this calculation were not available.
†Interclass correlation coefficient.
Data from Hakki et al: Circulation 63:1050-1055, 1981; Zoghbi et al: Circulation 73:452-459, 1986; Otto et al: J Am Coll Cardiol 7:509-517, 1986; Oh et al: J Am Coll Cardiol 11:1227-1234, 1988; Danielson et al: Am J Cardiol 63:1107-1111, 1989; Cannon et al: Circulation 71:1170-1178, 1985; Segal et al: J Am Coll Cardiol 9:1294-1305, 1987; Cannon et al: Am J Cardiol 62:113-116, 1988; Nishimura et al: Circulation 78:791-799, 1988; Desnoyers et al: Am J Cardiol 62:1078-1084, 1988; Tribouilloy et al: Am Heart J 128:526-532, 1994; Kim et al: Am J Cardiol 79:436-441, 1997; Goland et al: Heart 93(7):801-807, 2007; de la Morena et al: Eur J Echocardiogr 11(1):9-13, 2010; Furukawa et al: J Cardiol 59(3):337-343, 2012.
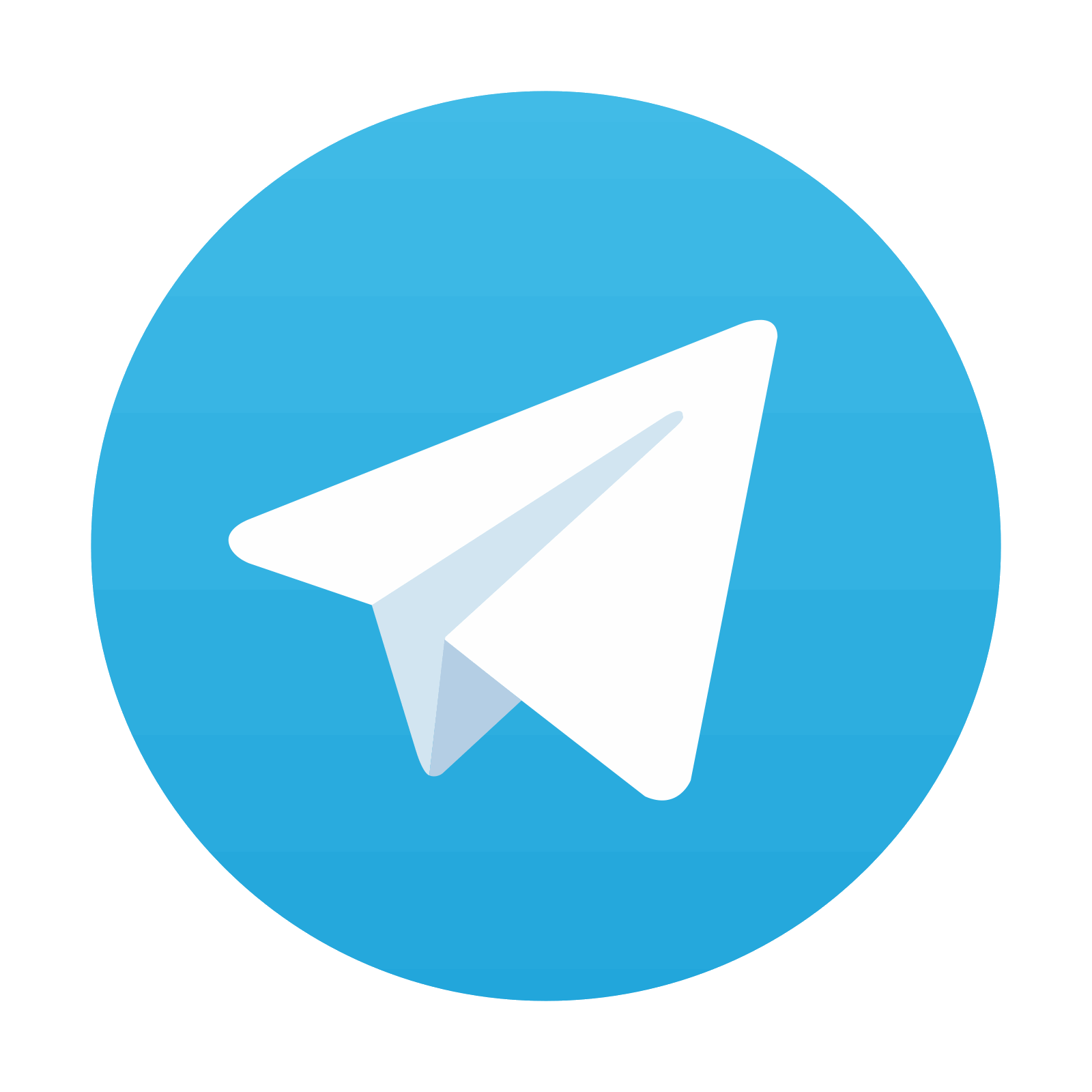
Stay updated, free articles. Join our Telegram channel
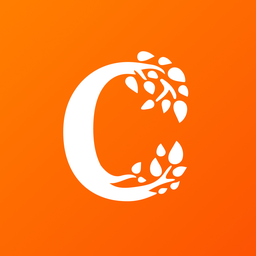
Full access? Get Clinical Tree
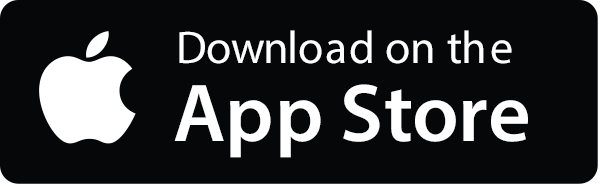
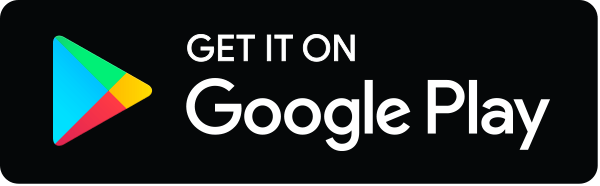
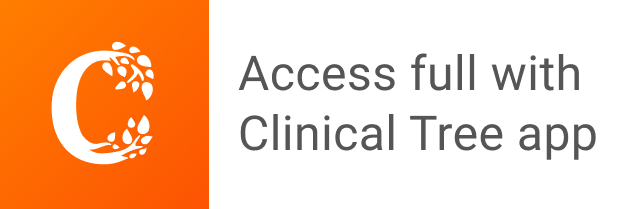