Key Points
- •
Atherosclerosis is a chronic immunoinflammatory disorder of the vascular, metabolic, and immune systems.
- •
Detection of subclinical atherosclerotic disease will enable initiation of earlier treatment and prevent the progression of atherosclerotic vascular disease and plaque disruption.
- •
MRI can evaluate various aspects of cardiovascular disease, including plaque morphology, vascular physiology, and myocardial function.
- •
High-resolution multicontrast MRI is used to characterize the specific tissue components of complex plaques, which aids in the detection of vulnerable plaque.
- •
Because of high reproducibility, MRI is valuable in assessment of plaque progression and regression.
- •
Molecular imaging elucidates the biology of various cellular and molecular targets in atherosclerosis before the development of gross phenotypic changes.
- •
MRI is an ideal molecular imaging modality because of its high spatial and temporal resolutions, noninvasive nature, lack of ionizing radiation, and excellent reproducibility.
- •
PET can assess inflammatory activity within atherosclerotic plaque because of avid FDG uptake and accumulation within the macrophages in inflamed plaque.
- •
PET can simultaneously evaluate cardiac perfusion and metabolism and hence plays a vital role in evaluation of selected patients with cardiovascular risk factors or disease.
Atherosclerosis is a diffuse, chronic immunoinflammatory disease of the vascular, metabolic, and immune systems characterized by deposition of lipid and fibrous products in the arterial wall. It is the result of complex biologic processes, resulting in various local and systemic manifestations after a long asymptomatic period. Genetic predisposition is part of the variability in development of atherosclerotic disease, plaque destabilization, and subsequent thrombosis.
Atherosclerosis is the single most important contributor to the burden of cardiovascular disease. In spite of advances in prevention, risk assessment, and treatment, cardiovascular disease is the major global cause of morbidity and mortality. An estimated 80 million American adults have cardiovascular disease, which accounted for 35% of deaths and an estimated cost of $475.3 billion dollars in 2009. The large population at risk for coronary events and frequent first presentation with a significant cardiovascular event or sudden death makes it seem reasonable to detect subclinical disease for initiation of earlier treatment to prevent the progression of atherosclerotic vascular disease and plaque disruption.
Conventionally, imaging has focused on detection and grading of stenotic lesions and perfusion abnormalities in organs distal to the stenosis. However, major acute events, such as myocardial infarction (MI) and stroke, are generally produced not by plaques with high-grade stenosis but by disruption of plaques that did not produce significant stenosis or perfusion abnormalities in stress tests, the so-called vulnerable plaque. In addition, recent evidence suggests that interventional treatment of coronary stenosis does not significantly improve MI and death rates in many persons with stable angina compared with a strategy of aggressive medical and lifestyle changes.
Hence, there is a need for new diagnostic tests that will be able to detect, quantify, and characterize the plaque, including its biologic activity and stability, ideally at an earlier subclinical stage in the disease process so that high-risk patients can be identified and treatment initiated earlier in the long asymptomatic phase to modify the disease progression and to reduce the risk of a major event. Because atherosclerosis is a diffuse disease, detection in one vascular territory implies involvement of other vascular territories, and treatment has to be both local and global. Novel therapeutic strategies include targeted transport vehicles allowing drug delivery to specific cells or cell structures.
Imaging of Atherosclerosis
The purposes of imaging in atherosclerosis are understanding of the natural history and pathobiology of atherosclerosis, diagnosis of subclinical disease and risk stratification, evaluation of plaque burden (location, size, chemical composition, biologic activity), and identification of vulnerable plaque. Serial imaging is performed to assess plaque progression or regression. Assessment of the impact of drug therapy on atherosclerosis directly is a surrogate endpoint for trials, reducing the need for expensive multicenter clinical trials or triaging which drugs go into expensive phase III clinical trials.
The ideal imaging technique should be sufficiently sensitive and specific for detection of atherosclerosis, inexpensive, reproducible, easy to perform, widely available, tolerated by patients, and noninvasive or minimally invasive. It should have no or minimal radiation, provide immediate results, quantify plaque components, be feasible in all vascular beds, add predictive value over measurement of established risk factors, and correlate highly with risks of subsequent major events.
The various available imaging options include invasive techniques, such as coronary angiography, intravascular ultrasound, palpography, and optical coherence tomography, and noninvasive techniques, such as high-resolution ultrasound, computed tomography (CT), single-photon emission computed tomography (SPECT), positron emission tomography (PET), and magnetic resonance imaging (MRI). Each has its advantages and disadvantages.
Magnetic Resonance Imaging
MRI uses the magnetic characteristics of the most abundant proton in the human body, hydrogen, to generate images. When they are placed in a powerful magnet, these protons align with the magnetic field; but on application of radiofrequency pulses, this alignment can be altered, causing the hydrogen protons to produce a varying magnetic field that can be detected by the scanner. The signal can be manipulated by multiple, additional magnetic fields for reconstruction of an image of the tissues of interest. The combination of radiofrequency and gradient waveforms used to obtain an image is called a pulse sequence. The appearance and signal intensity of any tissue on MRI depend on the type of pulse sequence and imaging parameters.
Rapid advances in the last 10 years have resulted in the emergence of MRI as an important tool in evaluation of cardiovascular disease. This is attributed to improved hardware, particularly the high–field strength magnets and high-sensitivity coils, and novel contrast agents, including those labeled with molecular targets. MRI is noninvasive and has no ionizing radiation; it has high inherent soft tissue contrast, spatiotemporal resolution, large field of view, and multiplanar imaging capabilities. Intravenous chelated gadolinium contrast material is useful for further characterization, but it is best avoided in patients with severe renal dysfunction because of the association with nephrogenic systemic fibrosis, a debilitating fibrosing condition believed to be secondary to an immune reaction to gadolinium particles deposited in the layers of skin, most probably due to high serum concentrations in patients with impaired renal function.
MRI Techniques in Cardiovascular Diseases
MRI plays an important role in the evaluation of various aspects of cardiovascular disease ( Table 28-1 ).
Technique | Assessment |
---|---|
MR angiography | Stenosis, dilation |
Whole-body MRA | Screening |
High-resolution MRI | Plaque detection, quantification, characterization |
Vascular physiology | Compliance, aortic stiffness with pulse wave velocity, flow-mediated vasodilation |
Functional data | Wall shear stress, neovascularization density |
Molecular imaging | Detection of cellular and molecular targets Assessment of vulnerable plaque |
Myocardial function | Volumes, mass, function Regional function |
Stress perfusion study | Ischemia |
Delayed enhancement | Viability |
Coronary MRA | Coronary luminal assessment |
Peripheral arterial MRI | MRA luminal assessment High-resolution MRI for plaque characterization Phosphocreatine kinetics |
Intravascular MRI | Plaque assessment and characterization Guidance for coronary intervention |
High-Resolution Multicontrast Imaging
High-resolution MRI is used to characterize the atherosclerotic plaque. The normal arterial wall has three layers, namely, the tunica intima, media, and adventitia, which cannot be separately distinguished on MRI. However, plaques are composed of variable quantities of cells, connective tissue, lipids, calcification, and debris, which can be evaluated by MRI ( Table 28-2 , Fig. 28-1 ).
Stage | Histologic Appearance | MRI Stage | MRI Appearance |
---|---|---|---|
I | Not grossly apparent; isolated macrophages with oxidized lipid droplets (foam cells) | I-II | Normal wall thickness; thin plaque, no calcification, <10% stenosis |
II | Fatty streak with multiple foam layers | ||
III | Preatheroma: raised fatty streak; multiple, small extracellular lipid cores; foam cells with lipid droplets; increasing smooth muscle cells | III | Diffuse intimal thickening; small eccentric plaque, no calcification |
IV | Atheroma: single, massive extracellular lipid pool, covered by proteoglycan-rich layer infiltrated with foam cells and smooth muscle cells with and without lipid droplet inclusion | IV-V | Plaque with lipid or necrotic core, covered by fibrous cap, possible small calcifications |
V | Fibroatheroma: type IV, but with a surrounding cap rich in fibrosis, possible small calcifications | ||
VI | Complex plaque with possible surface defect, hemorrhage, or thrombus | VI | Complex plaque with possible surface defect, hemorrhage, or thrombus |
VII | Calcified plaque | VII | Calcified plaque |
VIII | Fibrotic plaque without lipid core; fibrous tissue, no lipid core | VIII | Fibrous tissue, no lipid core, possible small calcifications |

Signals on MRI depend on proton density and T1 (longitudinal) and T2 (transverse) relaxation times of protons in water and fat. Differences in relaxation times of different tissues produce the contrast in MRI images, which can be manipulated by changing scan parameters or with contrast agents that modify relaxation times. MRI has high inherent soft tissue contrast that is ideal for characterization and differentiation of plaque components on the basis of biophysical and biochemical parameters, such as chemical composition, concentration, water content, physical state, molecular motion, and diffusion.
High-resolution MRI with multicontrast black blood techniques and dynamic contrast-enhanced angiography is used to characterize the specific tissue components of complex plaques, which aids in the detection of vulnerable plaque. High field magnets (1.5T or above) and phased array surface coils are used. Electrocardiography-gated axial images of the thoracic aorta are acquired in expiratory breath-hold. Multicontrast techniques use a combination of black and bright blood techniques that usually include T1 weighting, T2 weighting, proton density weighting, and three-dimensional time of flight, followed by postcontrast T1 weighting and time of flight.
Black blood images are acquired by the double inversion recovery fast spin-echo technique, which eliminates signal from flowing blood, resulting in high contrast between the dark blood and arterial wall with signal, enabling precise identification of the lumen-wall interface that is critical for assessment of plaque composition. T1, T2, and proton density weighting are achieved by use of different relaxation time (TR) and echo time (TE). Magnetization transfer and diffusion imaging are used in some protocols. Combinations of these different weightings help distinguish all the major plaque components, such as the lipid-rich necrotic core, fibrous cap, loose matrix, calcification, and intraplaque hemorrhage, all of which have different signal characteristics ( Table 28-3 ). The MRI findings have been validated in histologic studies and are highly reproducible both qualitatively and quantitatively, thus making MRI useful for serial evaluation of plaque progression or regression.
T1 | T2 | Proton Density | Enhancement | |
---|---|---|---|---|
Lipid | Hyper | Hypo | Hyper | None |
Fibrocellular | Hyper | Hyper | Hyper | None |
Calcium | Hypo | Hypo | Hypo | None |
Necrotic core | Low | High | High | None |
Fibrous cap | Low | Low | Low | Enhancement |
Hemorrhage | High | Low | Intermediate | None |
Vulnerable Plaques
Vulnerable plaque refers to thrombosis-prone plaques and plaques with high probability of undergoing rapid progression and thus becoming culprit plaques. The salient characteristics of vulnerable and stable plaques are listed in Table 28-4 .
Vulnerable Plaque | Stable Plaque |
---|---|
|
|
Minor | |
|
Large Lipid-Rich Necrotic Core
The large lipid-rich necrotic core is composed of extracellular lipids (cholesteryl monohydrate or unesterified cholesterol) and cellular debris. Necrotic core has low intensity on T2-weighted images because of short T2 and no contrast enhancement.
Thin Fibrous Cap
The fibrous cap is made up of intimal smooth muscle cells and connective tissue. Fibrous tissue has short T1, which results in bright signal on T1-weighted images and contrast enhancement. Rupture-prone plaques are very thin (65 to 150 µm), which is below the spatial resolution of MRI. In three-dimensional time of flight, the fibrous cap is seen at the interface of the bright lumen and dark wall, making it possible to assess its integrity ( Fig. 28-2 ). Higher strength magnets and multiple-channel coils may be necessary for further characterization. Easy visualization of a cap on MRI generally indicates that it is relatively thick. Nonvisualization (implying a thin cap) or disruption of the fibrous cap indicates potentially high risk plaques ( Fig. 28-3 ).


Active Inflammation
Inflamed plaques are characterized by rich and active macrophages, mast cells, T cells, proinflammatory cytokines, procoagulant mediators, increased matrix metalloproteinase expression, and reduced smooth muscle cells. Inflammation can be detected by contrast enhancement because of increased vascular permeability and edema or by molecular MRI techniques (see later).
Erosion or Fissure of Plaque Surface
Ulcerations are more common in symptomatic patients, regardless of the intensity of symptoms. In addition to the multicontrast black blood MRI, longitudinal black blood magnetic resonance angiography (MRA) increases the ability to identify ulcerations.
Superimposed Thrombus
Thrombus is more prevalent in symptomatic patients, especially with ipsilateral symptoms and plaque ulceration. Thrombotically active plaque associated with high inflammatory infiltrate is seen in 74% of patients presenting with major stroke. Thrombus is the plaque component that is more heterogeneous and difficult to detect.
Luminal Stenosis >90%
Shear stress imposes significant risk of thrombosis and sudden occlusion. It also indicates the presence of many nonstenotic or less stenotic plaques that are vulnerable for rupture and thrombosis.
Superficial Calcified Nodules
Calcified nodules within or close to the cap can protrude through and rupture the cap. Surface calcified nodules can have an exposed thrombogenic surface or can be encapsulated. Calcification (mainly calcium hydroxyapatite) has a low signal because of low proton density and diffusion-mediated susceptibility effects, making it difficult to be detected in black blood imaging, but it can be seen clearly in time-of-flight images.
Intraplaque Hemorrhage
Intraplaque hemorrhage is due to rupture of fragile microvessels. It has a variable signal, depending on the blood breakdown products. Methemoglobin, which is seen in the subacute stage, shows T1 shortening and high signal on T1 imaging. The window for observation of these changes is small, and it is essential to localize the hemorrhage within the plaque. Hemorrhages close to the lumen and intraluminal thrombosis are more often associated with embolism and symptoms than deep plaque hemorrhages are.
Outward Positive Remodeling
Vulnerable plaques are often associated with positive remodeling, which prevents luminal narrowing; as a result, the patient may be asymptomatic, and stress perfusion imaging is often normal. This is a surrogate marker of plaque vulnerability.
Adventitial Inflammation and Neovascularity
Adventitial inflammation and neovascularity are associated with high risk of plaque rupture. Gadolinium enhancement in T1-weighted MRI has high sensitivity for neovascularity (80%). Dynamic enhancement indicates vascular density, which is a marker of neovascularity, and delayed enhancement reflects increased permeability of inflamed vessels.
Post–Gadolinium Contrast Enhancement
Intense contrast enhancement indicates neovascularity and increased endothelial permeability, which results in entry of the contrast agent from blood plasma into the plaque. Strong enhancement is an indicator of plaque inflammation, which is a sign of vulnerability. The amount of enhancement is a marker of extent of acute inflammation. Late gadolinium enhancement is used to identify fibrous plaque.
Plaque Quantification
Plaque seen on black blood MRI has been validated with transesophageal echocardiography and with histopathology in animal models in multiple studies. Plaque can be measured manually or by automated techniques, such as MEPPS (morphology-enhanced probabilistic plaque segmentation algorithm) and CASCADE (computer-aided system for cardiovascular disease evaluation), which are based on probabilistic assumptions. The system determines the probability that each MRI pixel belongs to each of the four tissue types, namely, lipid, calcification, fibrous tissue, and loose matrix, and uses computing active contours to identify the boundaries of high probability regions of each tissue, which are then segmented and displayed in three dimensions. This reduces analysis time and reader bias and improves reproducibility. Quantitative measurements have been correlated with histology and are highly reproducible.
MRI of Individual Vascular Territories
The majority of the work on morphologic MRI of the atherosclerotic plaque has been performed in the carotid arteries and aorta because of their superficial location and large size, respectively. New studies are reporting assessment of plaques in coronary and other arterial territories, which are limited by small size and deep location. In all these territories, MRI acquisition takes a long time because of the need for high-resolution images.
Carotid Plaques
Black blood multicontrast MRI with surface coils has been well established and validated with histology in the demonstration of carotid plaque morphology (volume, area, and thickness), structure, and composition. There is a correlation between thinning or rupture of the fibrous cap and recent history of transient ischemic attack or stroke. Ipsilateral arteries in symptomatic patients have a higher prevalence of intraplaque hemorrhage and fibrous cap rupture. Intraplaque hemorrhage indicates an increase in plaque burden (increased necrotic core size), decreased lumen size, repeated intraplaque hemorrhage, and subsequent plaque rupture, making it a superior prognostic indicator ( Fig. 28-4 ).

A greater plaque burden and plaque eccentricity are prevalent among patients with prior major cardiovascular or cerebrovascular events. A prospective study of asymptomatic patients with a 50% to 79% stenosis showed that intraplaque hemorrhage, larger necrotic core area, and thinned or ruptured fibrous cap are associated with a high hazard ratio for subsequent clinical events ( Fig. 28-5 ). Increases in thickness of arterial wall, average area of intraplaque hemorrhage, and necrotic core area were associated with increased hazard ratio for subsequent clinical areas. MRI was also useful for evaluation of plaque regression in carotids (e.g., ORION [Outcome of Rosuvastatin treatment on carotid artery atheroma: a magnetic resonance Imaging ObservatioN] trial).

Aortic Plaque
Multiple studies have established a correlation between aortic plaques and coronary artery lesions. Thoracic aortic plaques are associated with hypercholesterolemia, and a correlation with low-density lipoprotein cholesterol (LDL-C) level has been shown. Abdominal aortic plaques are not associated with LDL-C levels, but they are associated with smoking. Although the exact reason for this variable susceptibility to different risk factors is not known, there are slight differences in the vascular beds. The abdominal aorta has less prominent vasa vasorum, resulting in nutrition only by diffusion, and it has higher blood pressures and higher stiffness with more collagen and less elastin and geometric tapering. Plaques in both the thoracic and abdominal aorta are associated with age and high blood pressure.
There is a high association between thoracic aortic plaques and coronary artery disease (CAD), more than with carotid or femoral artery plaques. Complex plaques are associated with cardiovascular events. Autopsy studies have shown a relationship between severe abdominal aortic plaques and cardiac events, but no association has been shown between CAD and abdominal plaques. An MRI study showed a higher prevalence of plaques in the thoracic (73%) and abdominal aorta (94%) in patients with CAD than in those without it. The extent of plaques correlated with the extent of coronary artery stenosis. Whereas the thoracic plaques were independently associated with CAD, the abdominal aortic plaques were not associated independently with CAD. There was no difference in prevalence between CAD with and without MI.
Complex plaques in the abdominal aorta were more prevalent in CAD patients with MI than without MI. Among patients without MI, complex aortic plaques were more prevalent in patients with complex coronary lesions than without complex coronary lesions. All these suggest that complex aortic plaques are linked to coronary plaque instability, which leads to development of MI and complex coronary lesions. Complex aortic plaque, especially in the abdominal aorta, is a good marker of coronary plaque instability. CT studies have also shown a better association between thoracic aortic plaques and CAD. Hence, thoracic aortic plaque is a better marker of coexisting CAD.
Coronary Artery Plaque
Plaque imaging in coronary arteries is hampered by the small diameter, tortuosity, deep location, and high mobility of the coronary arteries and the small volume of plaque. In addition, not all ruptured plaques cause acute coronary syndromes, and vulnerability is not static. A vulnerable plaque one day may look stable another day. There has been a good correlation between black blood MRI–measured coronary wall thickness and matched pathology sections, with good reproducibility. Increased wall thickness is seen in patients with more than 40% stenosis on angiography. Because of low resolution, MRI may overestimate coronary wall thickness in normal patients.
In asymptomatic type 1 diabetes mellitus, coronary wall MRI reveals higher plaque burden in subjects with nephropathy than with normoalbuminuria. Hyperintense plaque in T1-weighted sequences indicates an unstable plaque due to intraplaque hemorrhage, and it correlates with positive remodeling, ultrasound attenuation, lower Hounsfield units in CT, and transient flow after percutaneous coronary intervention. These findings are similar to those in carotid plaque characterization, which has been validated with histology. T1-weighted coronary plaque imaging has the potential for identification of complex coronary lesions in patients with unstable CAD.
High-resolution coronary plaque imaging has been performed in humans by 3T scanners with a 32-channel cardiac coil (0.5 × 0.5 × 3-mm resolution) and in animals by a 9T scanner (97-µm resolution) and intravascular MRI (117 × 156-µm resolution). A study demonstrated coronary arterial wall enhancement during acute MI due to edema and inflammation and resolution during delayed phase (6 months later), which offers the potential for visualization of inflammation in coronary atherosclerosis ( Fig. 28-6 ). However, enhancement was diffuse and not localized to the occlusive site.

MRI in Plaque Progression and Regression
Because of its high reproducibility, MRI is useful in the evaluation of plaque progression and regression and has been well established in numerous animal and human studies. The use of MRI has significantly reduced the sample size required for evaluation of pharmacologic regression of atherosclerosis in clinical trials. In an early study, with balloon-injured rabbits fed a high- versus low-cholesterol diet, MRI at 4 and 20 months showed higher wall thickness and stenosis in the high-cholesterol group. A similar MRI study with hypercholesterolemic rabbits fed a high-cholesterol diet for 12 weeks followed by a normal diet for 12 weeks showed increased wall volume during a high-cholesterol diet phase and decreased wall volume during the normal diet phase.
Numerous MRI studies have evaluated the response of carotid and aortic atherosclerotic lesions to various doses and regimens of medical therapy. MRI can measure plaque reduction and map changes in plaque composition in response to intensive lipid-lowering treatment and has been validated with histology. MRI has good reproducibility and low interscan variability and is able to detect a 10% change in wall volume and a 20% change in percentage of lipid-rich or necrotic core components.
In a study of asymptomatic subjects with 50% carotid stenosis, MRI showed that the rate of increase in carotid wall areas at 18 months was lower in those who took statins compared with the non-statin group. In a similar study of CAD patients, MRI showed significantly less lipid core area and lipid composition in patients who were receiving intensive lipid-lowering therapy compared with a nontreated group. MRI of the thoracic aorta demonstrated changes in plaque volume and lumen after simvastatin (20 to 80 mg) therapy for 6 months that correlated with LDL reduction. Other studies have shown continued decrease in plaques in the aorta and carotid arteries at 12 months and after 18 to 24 months of statin treatment, with minimal changes in luminal dimension.
A study comparing 5 mg versus 20 mg of atorvastatin for 12 months in hypercholesterolemic patients showed greater reduction of thoracic aortic plaque in the 20-mg than in the 5-mg group that correlated with reduced LDL-C levels. Abdominal aortic plaque did not change with the 20-mg dose but became worse with just 5 mg ( Fig. 28-7 ). Similar results were shown in a Japanese study that used 20 mg of atorvastatin. Another study that compared 20 mg versus 80 mg of simvastatin showed greater reduction of carotid and aortic plaque at 12 months in the higher dose group. All these studies prove that intensive atorvastatin therapy reduces LDL-C and causes plaque regression in thoracic aorta and carotid and coronary arteries.

Fibrates reduce triglyceride and increase high-density lipoprotein cholesterol (HDL-C) levels. An MRI study of hypertriglyceridemic patients after 400 mg of bezafibrate showed regression of thoracic aortic wall plaques without change in cross-sectional area, regression of abdominal aortic plaques with increase in cross-sectional area, and correlation between wall area change and triglyceride reduction and HDL-C increase. The change in abdominal plaques alone correlated with HDL particle size reduction and LDL size increase by nuclear magnetic resonance, implying that there might be different mechanisms in the thoracic and abdominal aorta for plaque regression and that triglyceride plays an important role in atherosclerosis in the abdominal aorta.
Because the abdominal aorta has no vasa vasorum and reverse lipid transport happens through diffusion into the lumen, there might be an increase in area after therapy, compared with the thoracic aorta, in which reverse transport occurs because of the presence of vasa vasorum and there is no significant reduction. MRI has also been used to compare the effects of statins versus statins plus the peroxisome proliferator activated receptor γ agonist on plaque.
Advances in Morphologic MRI
Higher field strength of magnets, dedicated multichannel phase array coils to increase signal-to-noise ratio, multislice motion-sensitized driven-equilibrium turbo spin-echo sequences to suppress plaque-mimicking artifacts, and three-dimensional isotropic sequences for evaluation of luminal surface and plaque definition are some of the latest advances in morphologic MRI. T2* imaging of iron forms within the plaque has demonstrated lower T2* values in symptomatic plaques.
High-Field MRI
3T MRI has the advantages of having twice the signal of 1.5T MRI and higher contrast-to-noise ratio, although it has higher chemical shift and susceptibility artifacts. Double inversion delay time is increased to accommodate increased blood T1. High spatial resolution images are acquired by parallel imaging and multiple averages, and these are useful for detection, characterization, and quantification of early and moderate plaques, with a high correlation between plaque quantity and components and histopathology. An 18-month follow-up study using 3T MRI showed 50% reduction in lipid content with minimal change in plaque size in a subject receiving aggressive statin therapy. Similar studies have been performed in coronary arteries, thoracic aorta, and femoral arteries. Higher field strengths, such as 7T and 9.4T, increase spatial resolution further and suggest potential for spectroscopic methods.
Coronary Magnetic Resonance Angiography
Coronary MRA provides information on the coronary artery lumen and vessel wall including plaque, which can be three-dimensionally reconstructed in multiple planes, without radiation or intravenous administration of contrast material. However, valuation of coronary arteries requires high spatial resolution (3 to 4 mm) and temporal resolution (<75 msec). A three-dimensional steady-state free precession whole-heart sequence with T2 preparation to decrease myocardial signal and fat saturation to decrease epicardial fat signal is used to image coronary arteries. Blood vessels appear bright because of intrinsic contrast. Images are acquired in diastole and expiration with free breathing technique using navigator gating of the diaphragm ( Fig. 28-8 ). To obtain high-resolution images (0.7 to 0.8 mm), acquisition time can be very long, up to 12 to 15 minutes. MRI has a sensitivity of 80% to 90%, specificity of >90%, and negative predictive value of 81% for identification of coronary artery stenosis. However, coronary MRA is not as widely used as CT angiography because of low sensitivity, long acquisition time, and lack of expertise in many hospitals.

Magnetic Resonance Angiography
Whole-body MRA can examine the entire arterial tree excluding the intracranial and coronary vessels in one sitting by use of bolus chase technique, multichannel receiver surface coils, and parallel imaging techniques, without radiation or arterial cannulation, and it is less nephrotoxic. Multistation techniques are used, typically a four-station technique; the first station covers supra-aortic arteries and the thoracic aorta, the second station covers the abdominal aorta, the third station covers the external iliac to popliteal areas, and the fourth station covers up to the ankle. Subsystolic venous compression in the calf can reduce venous contamination and increase signal-to-noise and contrast-to-noise ratios. Higher resolution scans can be performed at sites of plaques to characterize them and to quantify luminal stenosis. 3T magnet (higher signal), surface multichannel coils, parallel imaging, and blood pool agent (MS-325) will potentially improve the quality of MRA. MRA has been validated for pelvic and lower limb arteries. However, reproducibility has not been completely assessed yet, and it can overestimate stenosis.
The ability to image multiple vascular beds makes it useful in evaluation of atherosclerosis. MRA can be combined with whole-body MR screening. MRA has detected unknown arterial disease in different populations, such as those characterized by advanced age, diabetes, and post-MI. MRA is useful for repeated clinical examinations but has not yet been studied or validated to predict future cardiovascular events. An atherosclerotic score index (ASI) has been measured by scoring luminal narrowing (1, normal; 2, <25%; 3, 26% to 50%; 4, 51% to 75%; 5, 76% to 99%; 6, occlusion) in 40 extracardiac segments and dividing the sum by the number of analyzable segments ( Fig. 28-9 ). ASI was higher in patients with CAD than in those without and correlated with Framingham and PROCAM risk scores. An ASI >1.54 has 59% sensitivity, 86% specificity, and 84% positive predictive value for predicting CAD and confirming increased risk of CAD in those with extracardiac atherosclerosis, in addition to giving an estimate of total atherosclerotic burden in the body.

Peripheral MRI
Peripheral arterial atherosclerosis is the most common cause of peripheral arterial disease, often leading to vascular obstruction. MRA, high-resolution black blood imaging, and first-pass contrast-enhanced dual contrast perfusion imaging of the calf are the MRI techniques used in assessment of peripheral arterial disease. MRA can detect and quantify luminal stenosis, although it is technically challenging because of artifacts such as signal loss from in-plane saturation, turbulent flow, metallic clips and stents, and end-organ effects of peripheral arterial disease. High-resolution black blood imaging using surface coil and flow saturation can diagnose preclinical vascular disease, measure plaque volume, assess disease severity, and monitor plaque progression or regression in response to therapy.
The superficial femoral artery is the ideal vessel because it is superficial and nonmobile. Popliteal arteries have also been evaluated to demonstrate the changes that occur with remodeling and restenosis after angioplasty. First-pass contrast-enhanced dual contrast perfusion imaging of calf muscle at peak exercise with an MR-compatible pedal ergometer and 31 P MR spectroscopy at peak exercise measure phosphocreatine recovery kinetics, which varies greatly between normals and patients with peripheral arterial disease, particularly at maximal workload.
Myocardial Function
Global Myocardial Function
Left ventricular volumes, mass, and ejection fraction are important markers of cardiovascular disease and independent predictors of cardiovascular events, with changes seen before onset of symptoms. Left ventricular hypertrophy is considered an abnormal response to conditions such as hypertension, not physiologic or compensatory, and it is an independent predictor of cardiovascular events. Left ventricular mass is an important marker of subclinical disease and decreases after therapy reduces adverse events. The MESA study has demonstrated an 18-fold higher rate of congestive heart failure during a short period of observation in those with left ventricular hypertrophy, after accounting for traditional risk factors and coronary calcium score.
MRI is the most accurate technique in the evaluation of cardiac volumes and mass, with only 5% standard errors compared with 20% for echocardiography. Volumes are measured manually or semiautomatically by steady-state free precession sequences obtained in the short-axis plane, by drawing manual or automatic endocardial and epicardial contours. Mass is obtained by multiplying the wall volume with myocardial density (1.05 g/cm 3 ) ( Fig. 28-10 ). With increasing age, particularly in men, the mass-to-volume ratio increases, although left ventricular mass is maintained because of decrease of end-diastolic volume due to concentric remodeling.

Regional Myocardial Function
Regional changes often precede global abnormalities in cardiac function. Strain is fractional change in length from the resting state in diastole to contraction in systole. Radial, circumferential, and longitudinal strains can be measured on MRI by myocardial tagging techniques, such as SPAMM (spatial modulation of magnetization) or CSPAMM (complementary spatial modulation of magnetization), which produces dark saturation bands perpendicular to the scanning plane ( Fig. 28-11 ). Strain is quantified by harmonic phase analysis, which is more accurate than visual estimation of regional function. Strains can be measured separately in the subendocardial, mid-wall, and subepicardial layers. Other techniques for tagging are strain-encoded imaging (tags are parallel to imaging plane), displacement encoding with stimulated echoes (DENSE), and velocity encoding phase shifts with phase contrast imaging.

With age, although the ejection fraction is maintained in normal range, peak strain decreases, particularly in the left anterior descending territory of men. In women, strain increases with concentric remodeling in all but the highest quartile of mass-to-volume ratio. A direct relationship between regional diastolic dysfunction (decreased diastolic strain) and increasing left ventricular mass is demonstrated in asymptomatic individuals. After control for established risk factors, increased diastolic blood pressure was associated with decreased circumferential strain in asymptomatic individuals, particularly in smokers.
Stress Perfusion MRI
First-pass myocardial perfusion imaging with MRI is a sensitive technique to detect myocardial ischemia, with high detection of CAD compared with coronary angiography, and it has a 100% negative predictive value for subsequent CAD. Perfusion MRI is performed both at rest and after pharmacologic stress (adenosine or dipyridamole). Intravenous contrast produces T1 shortening of normal myocardium, resulting in bright signal on T1-weighted sequences. Hypoperfused areas (ischemic or revascularized infarct) appear dark ( Fig. 28-12 ). Microvascular dysfunction is seen as a subendocardial perfusion defect in a nonvascular distribution. Sequences used for perfusion are typically multislice T1-weighted two-dimensional sequences, which could be steady-state free precession (SSFP), fast low-angle shot (FLASH), or gradient-recalled echo planar imaging (GRE EPI), either inversion or saturation recovery based or hybrid, each with its advantages and disadvantages. Typically, perfusion studies are followed by delayed enhancement images to evaluate myocardial viability, and this enhances the performance of adenosine stress cardiovascular magnetic resonance. Detection of even small amounts of myocardial delayed enhancement in patients without known MI is an adverse prognostic indicator. Direct assessment of myocardial perfusion after adenosine stress is sensitive, whereas adenosine-induced wall motion abnormalities are highly specific.

Semiquantitative analysis of the blood flow could be obtained by analysis of the postcontrast myocardial signal intensity as a function of time. Myocardial perfusion reserve index is the ratio of the maximal upslope of the time-intensity profiles at stress and at rest, which is a good indicator of perfusion. After vasodilation in a normal vessel, there is a peak followed by washout before plateau. If there is no overshoot, it indicates no vasodilator response. Absolute quantification is possible if there is an accurate estimate of arterial input function, which is measured from the left ventricular blood pool signal. Dual bolus or dual sequence methods improve accuracy. Quantification of myocardial blood flow and myocardial perfusion reserve can be performed through deconvolution models or model-independent analysis. MRI has a higher accuracy for subendocardial than for transmural perfusion analysis.
Perfusion imaging can also be performed without intravenous contrast material by endogenous contrast mechanisms. In the BOLD (blood oxygen level dependent) technique, normally perfused areas have oxyhemoglobin, which is slightly diamagnetic, producing normal MRI signal, but hypoperfused areas have deoxyhemoglobin, which is paramagnetic and causes signal loss in T2*/T2-weighted images. ASL (arterial spin labeling) works by magnetically labeling blood flowing into slices of interest, which then exchanges with tissue water, altering the tissue magnetization. Subtraction of the image with labeled inflowing spins from an image without spin labeling gives a perfusion-weighted image.
Dobutamine MRI
Dobutamine infusion makes ischemic segments dysfunctional, which could be assessed by cardiac MRI as an alternative test for the diagnosis of CAD and quantifying myocardium at risk, particularly useful in patients in whom echocardiography cannot be performed. The sensitivity of dobutamine MRI is higher than that of echocardiography, and it can be further enhanced by myocardial tagging. It is also useful in predicting left ventricular functional recovery after coronary revascularization. Combined adenosine and dobutamine stress imaging showed 99% survival in patients with normal images and 84% with abnormal images in a series of 513 patients with known or suspected coronary disease during a mean follow-up of 2.3 years. In a multicenter, multivendor trial, perfusion cardiovascular magnetic resonance was proven to be a valuable alternative to SPECT for CAD detection, showing equal performance in the head-to-head comparison. It is also useful in assessing the results of a percutaneous coronary intervention.
Intravascular MRI
Intravascular MRI (IVMR) can be used to image small vessels, such as coronary arteries and iliac or renal arteries, with high signal-to noise-ratio, spatial resolution, and temporal resolution. Intravascular coils have been used for lesion assessment and characterization in carotid and iliac arteries, although there was not enough spatial resolution to visualize thin fibrous cap or atheroma thickness. IVMR can also evaluate increased lipid levels associated with potentially vulnerable plaques, with the help of an integrated, self-contained MRI probe, with magnets and transmit-receive radiofrequency coils on the tip of the catheter that measure lipid concentration in the arterial wall based on apparent diffusion coefficient (ADC). Fibrous tissue has high ADC (unrestricted diffusion), but lipid-rich tissue has low ADC (restricted because of large cholesterol esters).
Intravascular coils within the venous system can evaluate the adjacent arterial system. IVMR is potentially useful for guiding coronary interventions such as angioplasty and stent placement, with good real-time visualization of the catheter and three-dimensional multiplanar reconstruction capabilities and without need for fluoroscopy, radiation, or nephrotoxic contrast agents. IVMR is also used to confirm delivery of local gene therapy or nanoparticles to plaques or vascular wall by a special balloon catheter system. Limitations of intravascular systems are their invasiveness, catheter size, length of imaging protocols, and local heating.
Shear Stress
Plaque rupture is frequently seen at areas exposed to high wall shear stress, typically upstream to maximal stenosis. High wall shear stress also induces antiproliferative activity by endothelial cells on the upstream side, which enhances plaque vulnerability. Information on vascular geometry, inflow conditions, and plaque is acquired from MRI including angiography, and a computational fluid dynamic model is created from which shear stress acting on the luminal surface can be computed from the velocity field. It is unclear how variable geometric reconstruction and restricting assumptions on blood rheology or vessel wall compliance affect the accuracy of results. Wall shear stress can also be calculated semiautomatically and with good reproducibility with model-based segmentation of phase contrast MRI by determination of flow volume and maximal flow velocity in cross sections of these vessels ( Fig. 28-13 ). There is site-specific variation of wall shear stress based on this method.
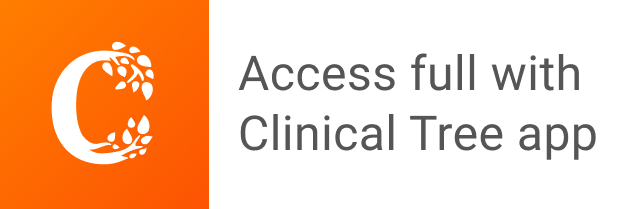