Ultrafiltration Therapies for Congestive Heart Failure
Maria Rosa Costanzo
Traditional diuretic therapies for congestion in heart failure patients are simultaneously ineffective and expensive. Approximately 1 million hospitalizations, with an estimated cost of $23 billion, occur annually in the United States due to decompensated heart failure (1,2). With an average hospital cost of $5,905 and reimbursement of $4,617, U.S. hospitals lose approximately $1,300 per heart failure admission (2,3,4). Data from the Acute Decompensated Heart Failure National Registry (ADHERE) show that the majority of heart failure hospitalizations are due to fluid overload in patients who have failed treatment with oral diuretics (5). Despite use of intravenous diuretics in 90% of patients, the average length of stay for a heart failure patient is 4.3 days and 42% of the patients are discharged without complete resolution of symptoms. With current treatment strategies, 50% of the patients lose ≤5 pounds from admission weight and 20% gain weight during the heart failure hospitalization (5). The failure to effectively resolve congestion and reduce weight may contribute to readmission rates, which may be as high as 50% at 6 months (6).
Although many heart failure patients can be success-fully treated with diuretics, 25% to 30% of patients develop diuretic resistance, defined as reduced diuresis and natriuresis before resolution of congestion (7). Causes include functional renal failure, hyponatremia, altered diuretic pharmacokinetics, and neurohormonally mediated sodium retention. In salt-restricted patients, fractional excretion of sodium decreases with continued exposure to loop diuretics, the braking phenomenon, and intermittent diuretic administration causes postdiuretic sodium retention (8). Therapies to ameliorate diuretic resistance, including fluid and sodium restriction, increased angiotensin-converting enzyme inhibitors (ACEIs) doses, use of diuretic combinations, and changes in the timing and route of diuretic administration, have limited success (9,10,11,12,13).
Finally, hospital stay may be inappropriately prolonged when aggressive use of intravenous diuretics leads to worsening renal function (14,15). Renal insufficiency and diuretic resistance worsen outcomes as well as hospital length of stay and cost of heart failure patients (16,17,18,19,20,21,22,23,24). A Study of Left Ventricular Dysfunction (SOLVD) analysis indicates that even mild renal insufficiency in patients with left ventricular systolic dysfunction independently predicts all-cause and pump failure mortality and the combined endpoint of death and heart failure hospitalizations (25,26). An increase of serum creatinine of >0.3 mg/dL, which occurs in nearly one-third of heart failure patients, is associated with poorer prognosis and longer hospitalization (11,27). Recently, the safety of diuretics in heart failure patients has been questioned (28,29). In 1,153 heart failure patients enrolled in the Prospective Randomized Amlodipine Evaluation (PRAISE), high diuretic doses independently increased mortality, sudden death, and pump failure death, associating diuretic resistance with a poor prognosis in heart failure patients (30). Intravenous diuretic use can decrease cardiac output, increase pulmonary capillary wedge pressure and total systemic vascular resistance, and reduce renal blood flow and glomerular filtration rates (31,32). The results of recently published studies have confirmed the association of high diuretic doses with worsening renal function in heart failure patients (33).
Pathophysiology of Sodium and Water Retention in Heart Failure
The data previously summarized are sobering, but not surprising, because in heart failure patients hemodynamic abnormalities and neurohormonal activation lead to progressive deterioration of renal function. Knowledge of the processes linking cardiac and renal dysfunction is critical for the understanding of the effects of mechanical fluid removal by ultrafiltration in patients with heart failure (Fig. 43-1) (34).
In heart failure, arterial underfilling produced by a decrease in cardiac output is the primary determinant of renal sodium and water retention. Because approximately 85% of the plasma volume resides in the low-pressure venous side and only 15% in the arterial side of the circulation, an expansion of total blood volume can occur in the venous system and yet arterial underfilling causes the kidney to increase reabsorption of sodium and water. The unloading of high-pressure baroreceptors in the left ventricle, carotid sinus, and aortic arch, resulting from arterial underfilling, is an afferent signal that decreases vagal and glossopharyngeal inhibitory tone in the central nervous system. This leads to activation of the sympathetic nervous system, which mediates an initially compensatory but later detrimental increase in peripheral and renal vascular resistance and increase in renin release from the kidney (35). Renin then activates angiotensin II, which stimulates secretion of aldosterone from the adrenal cortex, leading to renal sodium and water retention.
In addition, activation of the sympathetic nervous system stimulates the supra-aortic and paraventricular nuclei in the hypothalamus, which results in nonosmotic release of vasopressin and subsequent aquaporin 2-mediated renal water retention. As the left ventricle fails, the increase in left atrial and ventricular pressures stimulates release of natriuretic peptides, which mediate both vasodilatation and renal sodium excretion (36). Eventually, however, vasoconstrictive and volume-retaining mechanisms overwhelm the effects of natriuretic peptides. Initially, glomerular filtration rate is maintained by the increase in efferent arteriolar resistance and capillary hydrostatic pressure. Ultimately, however, renal blood flow decreases to the point where glomerular filtration rate cannot be maintained and renal dysfunction becomes progressive (37).
Salt and water retention in heart failure is further augmented by the development of diuretic resistance (7,8,9). The magnitude of natriuresis decreases after each diuretic dose (braking phenomenon). A decline in effective intravascular volume, by reducing the amount of sodium chloride (NaCl) that is filtered and by increasing that which is reabsorbed, is key to the occurrence of the braking phenomenon. When given acutely, thiazide diuretics reduce NaCl absorption in the proximal tubule by inhibiting carbonic anhydrase (38). In contrast, intravascular volume contraction due to chronic administration of thiazide
diuretics increases NaCl reabsorption both in the proximal tubule due to increased filtration fraction and decreased renal interstitial pressure, and in the distal tubule due to reduced delivery of NaCl (38).
diuretics increases NaCl reabsorption both in the proximal tubule due to increased filtration fraction and decreased renal interstitial pressure, and in the distal tubule due to reduced delivery of NaCl (38).
In addition, increased efferent sympathetic nerve activity reduces urinary NaCl excretion by reducing renal blood flow, by stimulating renin release at the macula densa, by enhancing tubular NaCl reabsorption, and by interacting with hormonal modulators of NaCl transport. Loop diuretics stimulate renin secretion by inhibiting NaCl uptake into the macula densa cells and by enhancing renal production of prostacyclin. Furthermore, chronic administration of loop diuretics induces distal tubular epithelial cells hypertrophy and hyperplasia due to increased solute delivery to the distal tubule (39,40).
In the milieu of heart failure and diuretic resistance, an attractive alternative to diuretics is removal of excess fluid with ultrafiltration, a therapy which does not cause prolonged intravascular volume contraction or stimulation of macula densa-mediated neurohormonal activation.
Ultrafiltration
The Process
The process of ultrafiltration consists of the production of plasma water from whole blood across a semipermeable membrane (hemofilter) in response to a transmembrane pressure gradient (Fig. 43-2) (41). The pressure gradient across ultrafiltration membranes is generated by the classic Starling forces, which include the hydrostatic pressures in the blood and in the filtrate compartments, and the oncotic pressure generated by plasma proteins. Hydrostatic pressure is determined by the blood pressure in the filtering device, generated by either the patient’s endogenous blood pressure or by an extracorporeal pump plus the siphoning effect of suction occurring in the ultrafiltrate compartment. The sum of these pressures generates the transmembrane pressure, driving the plasma water through the hemofilter exactly as Starling forces operate in a capillary bed (41). The correlation between ultrafiltration rates and transmembrane pressure gradient describes the filtering membrane’s hydraulic permeability (Kf) (Fig. 43-3). The correlation is linear within a certain range of pressures, beyond which it flattens due to the coating of the blood side of the membrane by plasma proteins (42,43).
Ultrafiltration Membranes
Ultrafiltration membranes can be characterized according to different parameters. The polymeric structure can be of natural (cellulosic) or synthetic origin. Cellulosic membranes are homogeneous and hydrophilic, whereas synthetic membranes have a finely porous structure. These characteristics result in specific interactions with electrically charged solutes or hydrophilic-hydrophobic molecules and influence the membrane’s hemocompatibility. Finally, membranes can be divided into “low-flux” or “high-flux” according to their hydraulic permeability. For extracorporeal ultrafiltration, synthetic high-flux membranes (made of polysulfone, polyamide, polymethyl-methacrylate, or polyacrylonitrile) are generally employed because of their excellent permeability (Kf >20 mL/hour × mm Hg × m2) and biocompatibility (43).
Hemofilters also vary according to the ultrafiltration technique for which they are employed. While standard hemofilters are used for ultrafiltration accompanying hemodialysis, clotting-resistant minifilters providing ultra-filtration rates between 2 to 10 mL/minute are used for isolated or slow, continuous ultrafiltration (SCUF) (44). When hydrostatic pressure exceeds oncotic pressure, the ultrafiltrate, consisting of water and nonprotein-bound low- and middle-molecular-weight solutes, passes through the semipermeable membrane into the ultrafiltrate space, while macromolecules (mostly proteins and cells) are retained. With isolated ultrafiltration, the solute is passively removed by accompanying the solvent flow (convective transport) (45,46).
In contrast, solute removed by dialysis diffuses down a concentration gradient. Small solutes readily move through membrane pores by diffusion or convection, whereas larger molecules can pass very slowly through membranes only by diffusion. Convection actually helps direct larger molecules through the pores so that with convection the transport rates for small- and middle-sized molecules are similar. In convective transport, the solute concentration in the ultrafiltrate is essentially equal to the solute concentration in the water component of the plasma on the blood side of the membrane (46). The sieving coefficient, which is the ratio between the concentration of the solute in the filtrate and in the blood, represents the permeability of the solute for that membrane. Ultrafiltration membranes have pores with molecular size cut-off so that molecules larger than the pores cannot pass through the membrane, even with convection. A sieving coefficient of zero indicates that the solute is completely rejected by the membrane (macromolecules and proteins), whereas a sieving coefficient of 1 indicates that the solute readily passes through the membrane. The sieving coefficient of sodium is essentially 1, indicating that the ultrafiltrate has a sodium concentration similar to that of the plasma. Protein-bound molecules, such as drugs, are retained by all membranes except for those used in plasmapheresis procedures (45,46,47). Therefore, the sieving coefficient of a drug is that of the fraction of the drug which is not bound to plasma proteins (47,48). Albumin, a negatively charged macromolecule, creates a Gibbs-Donnan effect at the blood-membrane interface, slightly decreasing the sieving of small cations and increasing that of small anions.
Vascular Access and Extracorporeal Pumps
The process of ultrafiltration is performed on blood extracted from the patient after cannulation of an artery or vein. The blood is then returned to the patient via a separate access to the venous circulation (41). While in end-stage renal disease ultrafiltration is performed with the puncture of an arterial-venous fistula, graft, or permanent catheter, in patients with volume overload ultrafiltration is carried out with a temporary access to the circulation. With arterial-venous access, an extracorporeal blood pump is unnecessary because the pressure gradient between the arterial and venous circulations is a force sufficient to drive the blood to the surface of the ultrafiltration membrane. For the arterial-venous approach, the femoral artery and vein are percutaneously cannulated with large-bore, low-resistance catheters to achieve the maximal blood flow for a given arterial-venous pressure gradient.
Because in the Hagen-Poiseuille equation blood flow is the dependent variable, any constriction in the extracorporeal circuit increases resistance and lowers blood flow. If an extracorporeal pump is used, a double lumen venous catheter is placed in the femoral or jugular vein, allowing extraction and return of blood with minimal recirculation. With this approach, pressure is the dependent variable, while flow is maintained constant by the pump (Fig. 43-4) (49).
Thus, production of ultrafiltrate can be modified by several methods. Techniques to manipulate hydrostatic pressure
on the blood side of the membrane include increasing systemic blood pressure, lowering resistance in the blood pathway, and inserting a blood pump proximally to the filter. Alternatively, suction (negative pressure) can be generated on the filtrate side to draw plasma water through the membrane. Mechanical fluid removal methods can be divided in isolated ultrafiltration techniques, hemofiltration, and ultrafiltration in conjunction with dialysis. While pure ultrafiltration is only a fluid-controlling method, all the others can simultaneously achieve some degree of blood purification. In blood-cleansing techniques, as ultrafiltration occurs the ultrafiltrate is replaced with clean fluid that dilutes solute concentration in the blood not yet driven through the filter. Based on frequency and duration, ultrafiltration techniques can be classified as acute or isolated, intermittent or continuous. With appropriate ultrafiltration rates, the extracellular fluid gradually refills the intravascular space and blood volume is maintained. If the ultrafiltration rate is too high, blood volume may decrease because intravascular volume depletion exceeds reabsorption of fluid from the interstitium into the vascular space.
on the blood side of the membrane include increasing systemic blood pressure, lowering resistance in the blood pathway, and inserting a blood pump proximally to the filter. Alternatively, suction (negative pressure) can be generated on the filtrate side to draw plasma water through the membrane. Mechanical fluid removal methods can be divided in isolated ultrafiltration techniques, hemofiltration, and ultrafiltration in conjunction with dialysis. While pure ultrafiltration is only a fluid-controlling method, all the others can simultaneously achieve some degree of blood purification. In blood-cleansing techniques, as ultrafiltration occurs the ultrafiltrate is replaced with clean fluid that dilutes solute concentration in the blood not yet driven through the filter. Based on frequency and duration, ultrafiltration techniques can be classified as acute or isolated, intermittent or continuous. With appropriate ultrafiltration rates, the extracellular fluid gradually refills the intravascular space and blood volume is maintained. If the ultrafiltration rate is too high, blood volume may decrease because intravascular volume depletion exceeds reabsorption of fluid from the interstitium into the vascular space.
Therefore, the three key factors for the removal of an adequate amount of fluid without hemodynamic compromise include accurate determination of the amount of fluid to be removed, optimization of fluid removal rate, and maintenance of circulating blood volume (50). To facilitate appropriate amounts and rates of fluid removal, instruments that monitor blood volume changes can be inserted into extracorporeal circuits during treatment (e.g., Crit Line, produced by Hemametrics, Inc., Salt Lake City, Utah). With online blood volume monitors, ultrafiltration can be slowed or arrested until complete intravascular refill has taken place (51). In peritoneal dialysis, an osmotically active substance, such as glucose, creates a hypertonic dialysate. This hypertonicity is the driving force for the ultrafiltration of plasma water from peritoneal capillaries across the peritoneal membrane into the peritoneal cavity, from which it is eventually drained away.
Key Differences in Fluid Removal with Diuretics, Ultrafiltration, and Dialysis
To understand the differential effects of diuretics, ultrafiltration, and dialysis on hemodynamic and neurohormonal abnormalities occurring in heart failure, it is critical to recognize the distinctive characteristics of fluid removal with these three therapies. The fluid removed with diuretics has a sodium concentration lower than that of the plasma. In contrast, the ultrafiltrate is essentially isoosmotic and isonatric compared with plasma, except for minimal differences due to the Gibbs-Donnan effect produced by albumin. Therefore, for any amount of fluid withdrawn, more sodium is removed with ultrafiltration than with diuretics (41). With diuretics, natriuresis drives water excretion and, therefore, sodium and water removal cannot be dissociated (38).
With hemofiltration techniques the ultrafiltrate is similar to plasma water, but total body sodium can be manipulated by adjusting sodium concentration in the replacement solution. The ability to dissociate sodium and water removal permits the normalization of electrolyte levels in both extracellular and intracellular compartments (41). With diuretics, intravascular volume contraction is prolonged and inhibition of NaCl uptake in the macula densa, coupled with augmented release of prostacyclin, enhances renal secretion of renin (41). These effects augment neurohormonal activation which, in turn, promotes sodium and water retention, ultimately reducing the diuretics’ ability to relieve the signs and symptoms of circulatory congestion (35,36,37,38,39,40,41,42,43,44,45,46,47).
In contrast, ultrafiltration does not stimulate macula densa-mediated neurohormonal activation, nor does it produce prolonged intravascular volume contraction because ultrafiltration removes fluid from the blood at the same rate at which fluid is reabsorbed from the edematous interstitium (Fig. 43-5) (52). In fact, changes in plasma volume (ΔPV) during ultrafiltration can be estimated from changes in hematocrit(Ht) according to the following formula:
ΔPV = 100/(100 – Htpre) × [100 (Htpre -Htpost)]/Htpost
where “pre” and “post” are the two time points considered. Plasma refilling rate (PRR, mL per minute), which represents a measurement of the fluid volume transport from the interstitium into the vascular space during ultrafiltration, is filtrate volume/time, where time is the duration of ultrafiltration if ΔPV = 0. When PRR equals the ultrafiltration rate, blood volume stability is preserved.
Ultrafiltration performed in conjunction with dialysis results in hypotonia or hypertonia because water removal is linked to that of other solutes such as urea and electrolytes, which are removed in proportions dependent upon the technique used. Diffusion removes solute osmoles preferentially from the vascular space and extracellular fluid. Because cells become hypertonic compared to extracellular fluid, water moves from extracellular fluid into the cells. Because dialysis also results in fluid removal across the dialyzer, extracellular fluid volume depletion and hypotension can occur. Because with filtration the osmolality of cells and extracellular fluid remains the same, as extracellular water and solutes are removed by the filter, cellular fluids and solutes quickly replenish the extracellular fluid space (53,54,55). Thus, the basis for maintenance of cardiovascular stability during ultrafiltration relates to many factors, including minimal osmolar shifts, appropriate neurohormonal sympathetic responses, and rapid redistribution of volume. These responses have been observed with nearly all continuous or intermittent ultrafiltration techniques (56,57,58,59,60,61,62,63,64,65,66,67,68,69,70,71,72,73,74,75,76,77).
The following description of individual techniques will be preceded by an overview of the effects of ultrafiltration in patients with varying acuity and severity of congestive heart failure.
Rationale for the use of Ultrafiltration in Heart Failure
The facts previously summarized indicate that the unique feature of ultrafiltration is the ability to remove excess fluid from the extravascular space without significantly affecting circulatory volume (Table 43-1). Thus, ultrafiltration techniques appear ideally suited to interrupt the vicious circle of volume overload, neurohormonal activation, and worsening renal dysfunction occurring in heart failure (34,62,64,65,66,67,78,79,80,81,82). In patients with both acute and chronic heart failure, ultrafiltration has been consistently shown to improve the symptoms of congestion, lower right atrial and pulmonary arterial wedge pressures, improve cardiac output, decrease neurohormone levels, correct hyponatremia, restore diuresis, and reduce diuretics requirements (Figs. 43-6 and 43-7) (69,83,84,85,86).
Table 43-1 Rationale for the Use of Ultrafiltration in Heart Failure | ||||||||
---|---|---|---|---|---|---|---|---|
|
Ultrafiltration in Moderate Heart Failure
The use of ultrafiltration in patients with only moderate heart failure has shed significant light on the mechanisms by which ultrafiltration improves hemodynamics and functional capacity and attenuates neurohormonal activation. Patients with moderate heart failure can have radiographic evidence of pulmonary congestion in the absence of peripheral edema. This pulmonary overhydration significantly contributes to decreased functional capacity and to mutually detrimental heart-lung interactions (87,88). Thus, a therapy that improves patients’ functional capacity by reducing pulmonary overhydration is attractive in patients with moderate heart failure.
In a controlled study in which 36 NYHA Class II and III heart failure patients were randomly assigned to either a single veno-venous ultrafiltration session removing 1,880 ± 174 mL of fluid, or no treatment, ultrafiltrated patients had significant reduction of right atrial pressure (from 8 ± 1 mm Hg to 3.4 ± 0.7 mm Hg; p <0.001) and pulmonary artery wedge pressure (from 18 ± 2.5 mm Hg to 10 ± 1.9 mm Hg; p <0.001); decreased radiographic lung water score (from 15.2 ± 2.2 mm Hg to 8.1 ± 1.0 mm Hg; p <0.001); as well as improvement in peak exercise oxygen consumption (VO2max) (from 15.5 ± 1 mL/kg per minute to 17.6 ± 0.9 mL/kg per minute; p <0.001). These changes were associated with increased maximal voluntary ventilation (from 110 ± 4 L/minute to 128 ± 9 L/minute; p <0.01) and tidal volume, and reduced dead space to tidal volume ratio at peak exercise (89). The improvement in exercise performance was associated with decreased norepinephrine levels at rest a downward shift in norepinephrine kinetics at submaximal exercise (suggesting normalization of neurohormonal responses during exercise), and an increase in norepinephrine levels during orthostatic tilt (suggesting normalization of circulatory responses to orthostatic hypotension) (89,90).
These favorable functional and neurohormanal changes persisted, on average, for 6 months after treatment. These data suggest that the observed benefit of ultrafiltration in patients with moderate congestive heart failure is attributable to decreased pulmonary stiffness resulting from a reduction in lung water content (89,91). This clearly improves lung mechanics, as suggested by changes in spirometry values both at rest and during exercise (87,89,92,93,94). Of 17 patients who underwent removal of 1,777 ± 35 mL of ultrafiltrate, compared to 8 patients without significant functional improvement, the 9 patients with a >10% increase in VO2max had a greater reduction in right and left ventricular filling pressures for a given cardiac output; increase in peak exercise ventilation due to
increased tidal volume and decreased dead space to tidal volume ratio; lower esophageal pressure swing (end expiratory-end inspiratory pressure) for a given tidal volume; and increased peak exercise dynamic lung compliance, defined as the ratio of changes in volume to changes in pressure over a tidal breath (from 0.10 ± 0.05 L/mm Hg to 0.14 ± 0.03 L/mm Hg; p <0.01) (87).
increased tidal volume and decreased dead space to tidal volume ratio; lower esophageal pressure swing (end expiratory-end inspiratory pressure) for a given tidal volume; and increased peak exercise dynamic lung compliance, defined as the ratio of changes in volume to changes in pressure over a tidal breath (from 0.10 ± 0.05 L/mm Hg to 0.14 ± 0.03 L/mm Hg; p <0.01) (87).
Thus, after ultrafiltration, ventilation not only increases but it also becomes more efficient. The pulmonary restrictive pattern commonly present in heart failure has been shown to result from increased extravascular fluid content, pulmonary blood volume, dead space to tidal volume ratio, and heart size (93,94,95,96). After ultrafiltration, reduction in intrathoracic pressure and removal of the constraining effect exerted on the heart by the overhydrated lungs decrease the external work of the heart (87,93,94). Improvement in pulmonary mechanics leads to a reduction in heart size and improvement of diastolic relaxation abnormalities. In 24 patients undergoing single veno-venous ultrafiltration of 1,976 ± 760 mL, reduction in filling pressures and extravascular lung water and improvement of VO2max were associated with decreased Doppler echocardiography signs of restriction, including a reduction in early to late filling ratio in both ventricles (mitral valve from 2 ± 2 to 1.1 ± 1.1; p <0.001, and tricuspid valve from 1.3 ± 1.3 to 0.69 ± 0.18; p <0.001) and an increase in the deceleration time of mitral and tricuspid flows, reflecting a redistribution of ventricular filling to late diastole. Change in ventricular filling patterns, lung water content, and functional performance persisted at 3 months.
These results suggest that reduction of interstitial lung water is the mechanism whereby ultrafiltration modified the pattern of ventricular filling and increased functional capacity. After ultrafiltration, the right and left ventricular Starling curves during exercise show a definite reduction in filling pressures for a given cardiac output. It seems likely that the overhydrated lungs exert a constraining effect on the heart and that normalization of lung water content can indirectly improve heart dynamics. The ultrafiltration-induced increase in dynamic lung compliance during exercise may have contributed to improvement of exercise performance by reducing the cost of breathing and to the fall of the external work of the heart, an organ which during its rhythmic action pulls and pushes against the lungs (97). These mechanisms are closely interrelated with reduction in lung water content, increase in dynamic lung compliance, and improvement in right and left ventricular Starling curves and exercise capacity. They provide a unified explanation why, after ultrafiltration-induced changes in the mechanical characteristics of the intrathoracic milieu, the heart functions in more favorable conditions and improvement lasts beyond the duration of treatment.
In heart failure patients, maximal flow-volume loops do not increase after exercise and functional residual capacity decreases at the onset of exercise and increases thereafter (98). It is possible that ultrafiltration may also improve these aspects of lung function. Lung diffusing capacity is strictly related to exercise capacity and is frequently impaired in patients with heart failure. Ultrafiltration does not improve lung diffusion (93). These data suggest that in chronic heart failure, impairment of alveolar capillary diffusion capacity is not due to lung water accumulation but to accumulation of cells and connective tissue along the alveolar capillary membrane. Because these changes are irreversible, changes in lung diffusion capacity are unreliable indicators of the efficacy of ultrafiltration.
The result of another study showed that the favorable hemodynamic, neurohumoral, and ventilatory responses to ultrafiltration occurring in stable chronic heart failure patients were not observed in patients who received only a continuous diuretic infusion matched to achieve equivalent fluid removal. Sixteen NYHA Class II and III heart failure patients were randomly allocated to receive either a single ultrafiltration treatment (n = 8) or intravenous furosemide (n = 8, mean furosemide dose = 248 mg) to remove approximately 1,600 mL of fluid (92). Soon after fluid withdrawal by either method, biventricular filling pressures and body weight were reduced and plasma renin, norepinephrine, and aldosterone levels were increased. After furosemide, neurohormones levels remained elevated for the next 4 days and during this period patients had positive water balance, recurrent elevation of filling pressures, and lung congestion without improvement of VO2max. In contrast, after ultrafiltration, neurohormones levels fell below baseline within 48 hours, whereas water metabolism was equilibrated at a new set point (less fluid intake and diuresis without weight gain) (Fig. 43-8). Favorable circulatory and neurohormonal changes were correlated with lung water reabsorption, which was increased only in ultrafiltration-treated patients. Improvement was sustained at 3 months after ultrafiltration (Figs. 43-9 and 43-10) (92).
These data indicate that while ultrafiltration and furosemide are equally effective in terms of acute volume of fluid removed and resolution of congestive symptoms, their long-term effects are significantly different. Specifically, the effects of ultrafiltration on pulmonary water metabolism and neurohormone levels are due to mechanisms not occurring with diuretics. The fluid removed by ultrafiltration has different sodium content compared to the fluid removed with diuretics. Indeed, ultrafiltration removes fluid with a sodium concentration similar to that of plasma, so that approximately 150 mmol of sodium are withdrawn with each liter of ultrafiltrate. In contrast, the urine of heart failure patients is hypotonic compared with
plasma and the 50 mmol of sodium usually present in 1 L of urine increases to only 100 mmol with furosemide administration (99). As a consequence, ultrafiltration provides the greatest possible amount of sodium elimination per unit of fluid removed. It is possible that the different amounts of sodium removed with similar amounts of fluid account for the differential effects of ultrafiltration and diuretics on neurohormonal responses, which in turn, will result in different renal sodium and water reabsorption.
plasma and the 50 mmol of sodium usually present in 1 L of urine increases to only 100 mmol with furosemide administration (99). As a consequence, ultrafiltration provides the greatest possible amount of sodium elimination per unit of fluid removed. It is possible that the different amounts of sodium removed with similar amounts of fluid account for the differential effects of ultrafiltration and diuretics on neurohormonal responses, which in turn, will result in different renal sodium and water reabsorption.
It should be noted that ultrafiltration’s clinical benefits are amplified and sustained over time in patients receiving ACEI. In one study, ACEI-treated patients maintained the weight reduction achieved after ultrafiltration, whereas weight returned to pre-ultrafiltration values in patients not taking ACEI (100). Improvement of exercise capacity and reduction in pulmonary interstitial fluid scores lasted longer after ultrafiltration in patients treated versus those not treated with ACEI. In chronic heart failure patients, the body’s defense reaction against hypovolemic stimuli is maintained, albeit blunted, by the renin-angiotensin system (101). Withdrawal of excess of fluid by ultrafiltration produces greater benefit when combined with ACEI because these drugs will block the neurohormonally mediated increase in renal sodium and water reabsorption occurring in response to forced dehydration. Indeed, it is well-known that ACEI action is optimal when the renin-angiotensin system is maximally activated (102). By inducing transient hypovolemia with the resulting transient activation of the renin-angiotensin system, ultrafiltration creates the ideal condition for ACEIs to exert their favorable hemodynamic and renal effects. This indicates that fluid balance is readjusted at a different set point in patients receiving ACEI.
Ultrafiltration in Decompensated Heart Failure
Ultrafiltration has been consistently shown to improve signs and symptoms of congestion, increase diuresis, lower diuretic requirements, and correct hyponatremia also in patients with advanced heart failure (69). Among 32 NYHA Class II–IV heart failure patients with varying degrees of hypervolemia, the baseline 24 hour diuresis and natriuresis were inversely correlated with neurohormone levels and renal perfusion pressure (mean aortic pressure-mean arterial pressure). The response to ultrafiltration ranged from neurohormonal activation and reduction of diuresis in patients with the mildest hypovolemia and urine output >1,000 mL per 24 hours to neurohormonal inhibition and potentiation of diuresis and natriuresis in those with the most severe volume overload and urine output <1,000 mL per 24 hours (69).
In the majority of cases, the decrease in norepinephrine level was proportional to the potentiation of diuresis. This finding suggests that subtraction of total body water uncovers enough cardiac reserve to increase cardiac output and attenuate neurohormonal activation. These benefits are then maintained because the resulting enhancement of diuresis leads to improved norepinephrine clearance from the circulation (84). As in moderate heart failure, it is possible that also in advanced heart failure the earliest effect of ultrafiltration is the reduction of the extravascular pulmonary fluid, with a subsequent decrease in pulmonary extravascular resistance, improvement of ventilation and gas exchange, and a decrease in hypoxia-induced vasoconstriction. Ultrafiltration itself via baroreceptor-mediated reflexes may reset neurohormonal activation, which, in turn, may explain the intermediate- and long-term benefits observed after ultrafiltration (84).
The removal of myocardial depressant factors (85) has also been invoked as an explanation for the clinical benefits associated with ultrafiltration. A myocardial depressant substance has been extracted from the ultrafiltrate obtained from patients with congestive heart failure and acute renal failure (37,103). In 36 patients with acutely decompensated heart failure, ultrafiltration was associated with an increased cardiac index and oxygenation status, decreased pulmonary artery pressure and vascular resistance, as well as reduced requirement for inotropic support (104). SCUF or daily ultrafiltration has also been shown to effectively control volume in patients with severe congestive heart failure regardless of concomitant renal function (34). It is not known if the clinical benefits of ultrafiltration translate into improved survival.
Acute heart failure, which is most commonly due to decompensation of chronic heart failure, can also occur in the setting of circulatory collapse complicating myocardial infarction, hypertension, pericardial disease, cardiomyopathy, myocarditis, pulmonary embolus, or arrhythmias or after cardiac surgery (105,106,107). In these clinical settings ultrafiltration has been used predominantly after diuretics have failed or in the presence of acute renal failure. However, the results of some studies suggest that earlier utilization of ultrafiltration can expedite and maintain compensation of acute heart failure by simultaneously reducing volume overload without causing intravascular volume depletion and re-establishing acid-base and electrolyte balance (39).
The use of ultrafiltration in patients with advanced heart failure has also highlighted the risks associated with mechanical fluid removal. Overly aggressive ultrafiltration in patients with decompensated heart failure can convert nonoliguric renal dysfunction into oliguric failure by increasing neurohormonal activation and decreasing renal perfusion pressure, with minimal opportunity of recovery of renal function. Patients with this outcome become permanently dependent on dialysis. In addition, permanent renal loss can eliminate the option of heart transplantation in patients with end-stage heart failure. Prior to initiating a treatment course with extracorporeal therapies, the clinician must clearly explain the risk and potential pitfalls of this technique. It is generally disastrous to add a chronic need for dialysis to an already decompensated cardiac status. In some cases a trial of ultrafiltration can be offered for a limited time to evaluate potential benefits. However, there must be a clearly delineated plan to withdraw support if predefined parameters of improvement are not achieved (108,109,110,111,112,113,114,115). Severity of renal dysfunction influences the type and intensity of ultrafiltration approach. Heart failure patients without overt renal impairment may benefit from isolated ultrafiltration. In patients with moderate to severe renal dysfunction, stabilization of cardiac and metabolic functions can only be achieved with hemofiltration (108).
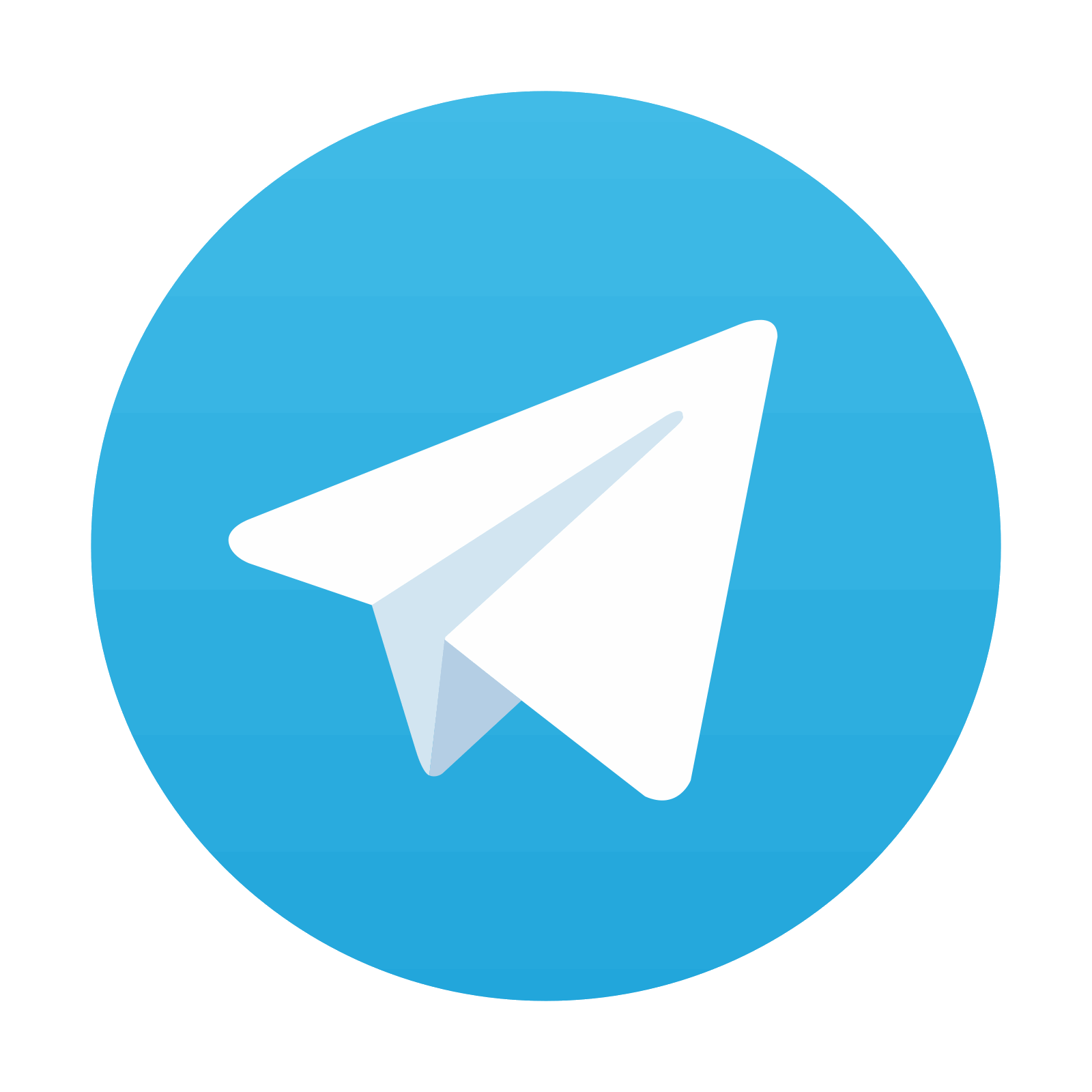
Stay updated, free articles. Join our Telegram channel
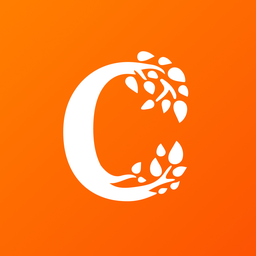
Full access? Get Clinical Tree
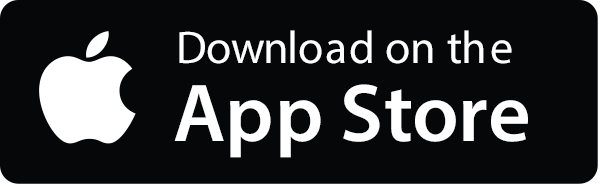
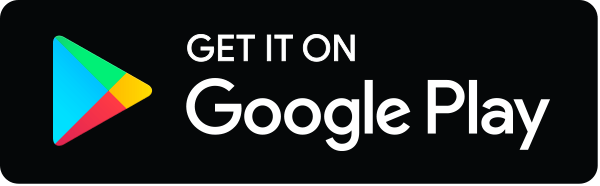