© Springer International Publishing AG 2017
Lynn M. Schnapp and Carol Feghali-Bostwick (eds.)Acute Lung Injury and RepairRespiratory Medicine10.1007/978-3-319-46527-2_33. Transgenic Animal Models in Lung Research
(1)
Division of Pulmonary and Critical Care Medicine, Department of Medicine, Center for Lung Biology, University of Washington, Seattle, Washington, USA
Keywords
Transgenic animal modelsCre recombinaseLung injury and developmentIntroduction
Advances in molecular biology have enabled the use of genetically modified mammalian vertebrates in human disease research. Due to their physiological similarity to humans, relative cost-effectiveness, and ease of husbandry, rodents have become the most widely used transgenic animals in modeling human disease. The goal of this chapter is not to undertake an exhaustive review of the history of transgenic animals, but rather to highlight recent advances in animal models used in pulmonary research and to offer practical guidance in the use of these transgenic animals. Over the past decade, the development of Cre-loxP technology has enabled the development of sophisticated transgenic animal models to address complex questions about development and function. While this technology is a powerful tool, many considerations need to be made when using these transgenic models. This chapter will give a brief overview of this technology as well as practical considerations in the use of these transgenic animals.
Transgenic Mice in Lung Injury Studies
Ever since the ability to introduce genetic modifications into mammalian embryonic stem cells was discovered in the 1980s, transgenic mice have assumed an integral role in the laboratory study of human disease [1]. Manipulations that result in gain-of-function and loss-of-function animal models have been used extensively over the last three decades to study biological pathways in lung pathogenesis. Heritable gene knock-ins and knockouts have been traditionally used to model human disease. Baron et al. [2] summarized genetically modified strains in their review of transgenic animals in lung biology research. For example, genetic knockouts of surfactant protein D and tissue inhibitor of metalloproteinases-3 lead to spontaneous histological changes compatible with chronic obstructive pulmonary disease [3, 4]. Models in which genetic manipulation does not lead to an overt phenotype but confers susceptibility to or protection against an injurious agent have also been widely used. For example, metalloproteinase-12 knockout mice have no observable lung phenotype during embryogenesis or in adulthood, however they are protected against emphysema with cigarette exposure [5]. These studies suggest metalloproteinases play a complex but important biological role airway inflammation, remodeling, and development of emphysematous changes.
Use of transgenic mice with global gene disruption has its limitations. Global knockout or overexpression of a gene may disrupt development and lead to embryonic or postnatal lethality. Conversely, biological pathways in pathogenesis may differ between species. Genetic manipulation in mice may not adequately recapitulate human disease. For example, attempts to generate a mouse model of alpha-1 antitrypsin (A1T1) deficiency have been unsuccessful to date. Depending on the mouse genetic background, serine protease expression in mice is controlled by multiple genes in the serpin family (Serpina1) whereas in humans, A1T1 expression is limited to one gene. Attempts to generate a knockout in one of the serine protease genes in mice (Serpina1a) have not been successful to date, with the unexpected discovery that Serpina1a-/- leads to embryonic lethality [6]. While human A1T1 expression is only controlled by one gene, Serpina1 null genotypes have been reported in humans suggesting A1T1 is not essential in human embryogenesis in contrast to mice. Given the important limitations seen in the generation of global knockout transgenic animals, other genetic manipulation strategies are required to study individual biological pathways in animal models. Recent advances in technologies that allow temporal and spatial control of genomic manipulation in mouse models are paving way to elegant transgenic models that address some of the limitations encountered in global knockout models.
Cre recombinase
Genomic manipulation using site-specific recombinases has become increasingly popular in transgenic mouse models. Recombinases are enzymes that serve a host of vital biological function in viruses and yeast. They recognize specific DNA sequences on the genome and mediate rearrangement of DNA with high efficiency, enabling molecular phenomena such as viral genomic integration and excision. The Cre recombinase derives from bacteriophage P1 and mediates recombination at specific 34 base pair sequences termed loxP sites [7, 8]. Of the many recombinases known, Cre recombinase requires no additional accessory proteins or specific substrate topology to mediate recombination efficiently at loxP sites [9, 10]. For these reasons, the Cre-loxP system has been widely used in transgenic mouse models to modify gene expression. Another well-studied recombinase with applications in transgenic animal studies includes the yeast recombinase Flp from Saccharomyces cerevisiae [11]. Application of these recombinases has enabled a host of novel studies in lung research including developmental studies, cell-fate mapping, and spatial or temporal gene disruption duties.
The 34 bp loxP sequence is directional. When two loxP sites are inserted into the host genome or linearized DNA in a head-to-tail fashion, Cre will mediate recombination between the loxP sites leading to the excision of the DNA between the loxP sites as a circular DNA plasmid [12]. In transgenic models, loxP sites may be introduced into targeted coding sequences in a specific gene (called a “floxed” gene). When crossed with a transgenic animal that expresses Cre recombinase under the control of a specific gene promoter, the bitransgenic progeny will undergo deletions of the floxed coding sequences where Cre recombinase is expressed. Such deletions may result in frameshift mutations or truncations during mRNA transcription, leading to null phenotypes.
Applications of Cre-loxP System in Animal Models
Studies in lung development and regeneration. The ability to manipulate the mouse genome using Cre-loxP transgenes was first demonstrated by Lewandoski and Martin [13] in 1997. The introduction of Cre-loxP technology in transgenic models has enabled a number of exciting discoveries and refinements in our understanding of cardiopulmonary development and regeneration. In these studies, transgenic animals expressing Cre under the control of a developmental gene promoter are crossed to transgenic animals carrying a reporter construct under the control of a ubiquitous promoter such as Rosa26. These constructs contain a STOP codon flanked by loxP sequences, and a chemical or fluorescent reporter downstream such as LacZ or any one of the fluorescent proteins (e.g., green fluorescent protein, tdTomato, yellow fluorescent protein) [14]. The result is constitutive silencing of the reporter until the floxed STOP codon is excised in the presence of Cre recombinase. In the presence of Cre recombinase, cells and their daughter cells constitutively and heritably express the reporter construct. Bitransgenic animals expressing Cre under a specific gene promoter (e.g., a gene of interest expressed only during embryonic development in a specific progenitor cell type) and the Rosa26-STOPflox/flox-reporter transgene allow investigators to map the fate of early progenitor cells by visualizing labeled cells and their daughter cells in the developing lung. The ability to identify the temporal and spatial fate of lung progenitor cells allows for more detailed examination of the pathways essential to the proper differentiation of the many lung cell types and improved understanding of disease states. Similarly, whereas the fully developed adult lung remains fairly quiescent, adult progenitor populations are present and may reenter cell cycle to repair and/or regenerate damaged cells following experimental injury stimuli. While the composition of and pathways utilized by these putative adult progenitor cells remain unclear, Cre-loxP technology has enabled a number of studies directed at identification of the pools of progenitor cells in the adult lung. For example, labeling of differentiated type II alveolar epithelial cells has shown that they are capable of acting as stem cells to repair damaged alveolar epithelium following lung injury [15]. Furthermore, this technology has been used to identify the progenitors of lung myofibroblasts following experimental lung injury and to expand on the field’s understanding of the key cellular effectors in fibrosis and lung repair [16, 17].
Spatial deletions. Another powerful application of Cre-loxP technology is its use in cell-specific deletion of genes. By carefully selecting cell type-specific promoters, investigators are able to engineer transgenic mice with targeted gene deletions in restricted populations of lung cells. These studies have led to a more refined understanding of the functional heterogeneity of different cell types in lung injury and repair. For example, an area of intense debate within the lung fibrosis field has been whether bone-marrow-derived, circulating progenitor cells termed “fibrocytes” directly contribute to collagen I deposition in lung fibrosis. These cells are CD45+ and display collagen IαI gene and protein expression. Furthermore, they localized to areas of fibrosis in experimental models of lung injury by immunohistochemistry [18] However, conflicting data have shown no evidence of CD45+ cells in areas of fibrosis by lineage-tracing studies [16, 17], calling into question the importance of fibrocytes in lung fibrosis. Kleaveland et al. further elaborated on these findings and examined collagen I production by fibrocytes in experimental lung injury. In their study, transgenic mice expressing Cre under a hematopoietic cell lineage promoter, Vav-Cre, crossed to a collagen IαI floxed transgenic animal were used to disrupt collagen IαI expression specifically in fibrocytes, without affecting fibrogenic cell types of non-hematopoietic lineages. Despite the inability of fibrocytes to transcribe the collagen IαI gene, collagen I deposition was unaffected in experimentally induced lung fibrosis. Therefore, the authors concluded fibrocytes did not functionally contribute to collagen I deposition [19]. Without the ability to restrict the deletion of the collagen IαI gene in fibrocytes, this type of study would not be possible. Another advantage of spatial deletion in gene disruption studies is that global knockout of a gene of interest sometimes leads to an embryonic lethal phenotype, rendering the model unsuitable for studies requiring adult animals.
Temporal Cre activation and deletions. A related application of the Cre-LoxP system involves the conditional activation of Cre in a temporally controlled manner. To achieve this, various constructs of Cre recombinase fused to ligand-binding domains have been generated, resulting in Cre recombinase (tethered to a ligand-binding domain) that is sequestered in the cytosolic compartment and unable to mediate genomic recombination at target loxP sites. Upon ligand exposure and binding to the ligand-binding domain (typically exogenously administered at the desired time point), Cre recombinase translocates to the nucleus to mediate genomic recombination. The most commonly used conditionally activated Cre recombinases involve mutated estrogen receptors and the administration of tamoxifen to liberate the Cre recombinases. Various widely available constructs include CreER, CreERT2, and MerCreMer. The ability to temporally control Cre activation enables studies in which timed recombination of the target, floxed gene is desired. For example, conditional knockout of a gene critical in development can be studied in adults using the CreER technology by administering tamoxifen to adults, thus bypassing the developmental stage where the gene’s function is vital.
Inducible Cre beyond tamoxifen. The doxycycline system was developed to reversibly suppress or activate gene expression in transgenic mice. This transgenic model consists of two transgenic lines bred to each other: (1) an activator line expressing either the tetracycline activator (TA, or tet-off) or the reverse tetracycline responsive transactivator (rtTA, or tet-on) and (2) an operator line with the desired transgene under the control of the tetO operator. In bitransgenic animals containing the activator and operator transgenes, doxycycline administration allows tetracycline activator to bind to tetO, suppressing transgene expression downstream (tet-off), or rtTA to bind to tetO, activating transgene expression (tet-on). This model was developed separately from the Cre/lox model to study gene expression in mouse models. Combining the Cre/lox system with tetO system has allowed for sophisticated methodolgies in doxycycline-dependent, cre-mediated gene manipulations. The generation of triple transgenic mice with rtTA expression under a cell-specific promoter, TetO-Cre, and loxP sites flanking a gene of interest result in animals that will have deletion of a gene of interest in a cell-specific manner when they are exposed to a diet with doxycycline. Perl et al. used this strategy to generate a triple transgenic animal combining rtTA expression under the SP-C promoter for epithelial tissue specificity, tetO-Cre, and CMV-loxP-LacZ-loxP-AP (ZAP) or CMV-loxP-LacZ-loxP-GFP (ZEG) reporter construct [20]. Following doxycycline exposure, these mice demonstrated reporter expression (alkaline phosphatase or GFP) in distal lung epithelial cells.
In addition to doxycycline, other inducers have been described. The use of a Cre fused to a progesterone receptor ligand-binding domain was first described by Kellendonk et al. [21]. In these transgenic mice, Cre activity is induced by the administration of RU-486. However, transgenic mice using this fusion Cre showed variable “leaky Cre” activity in which Cre activity was observed in unexposed transgenic animals (i.e., no RU-486) [22, 23]. Partly due to the leaky Cre phenomenon, inducible Cre transgenic lines using progesterone receptor ligand-binding domain fusion are less widely available compared to the tamoxifen-inducible CreER or CreERT2 counterparts.
Novel inducible Cre lines are under development to harness advances in pharmacologic stabilization of destabilized proteins. This technology utilizes mutant destabilizing proteins from humans or bacteria fused to a protein of interest. When the protein of interest is fused to the destabilizing protein, the complex is usually degraded by proteosomes. However, this degradation can be blocked by administration of a pharmacologic stabilizing agent. For example, Cre recombinase has been fused with the destabilizing dihydrofolate reductase (DHFR) from Escherichia coli to generate an inducible Cre transgenic animal that targets genes specifically in neurons [24]. In this model, the administration of trimethoprim (TMX) stabilizes the Cre-DHFR fusion protein driven by a neuron-specific promoter and leads to efficient Cre-mediated recombination in neurons. The advantage of TMX-inducible system includes ease of administration in animal models, high penetrance in most tissues, and lack of endogenous targets in mammals. This may prove to be a highly attractive model in lung research, but specific transgenic lines have yet to be developed and characterized for lung biology.
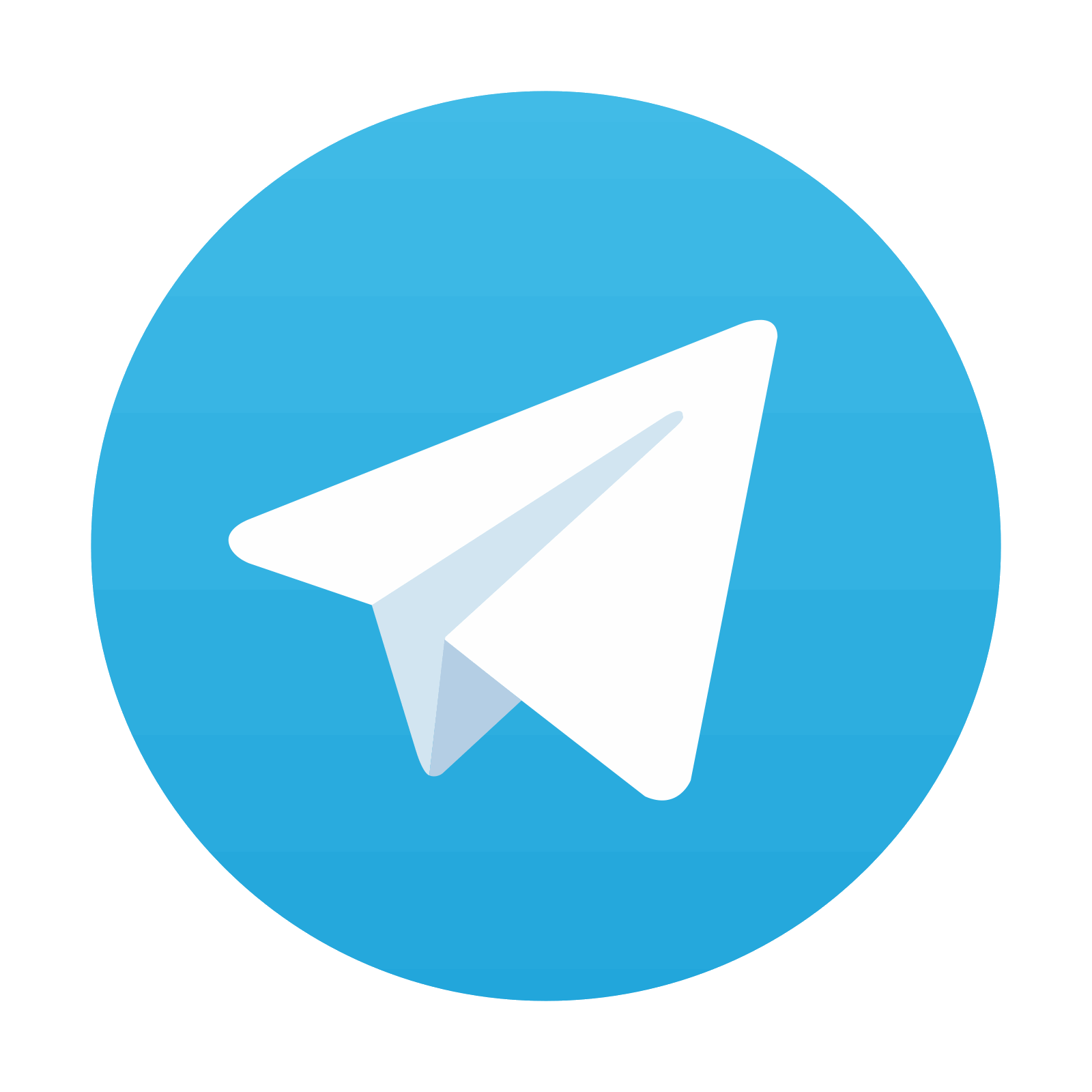
Stay updated, free articles. Join our Telegram channel
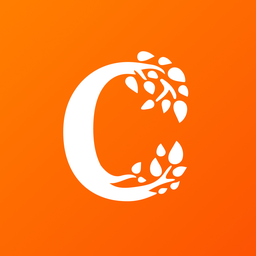
Full access? Get Clinical Tree
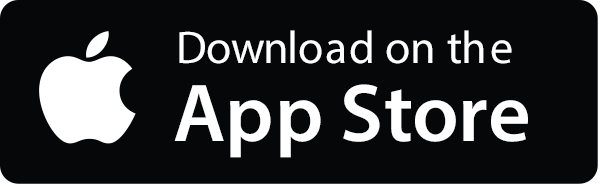
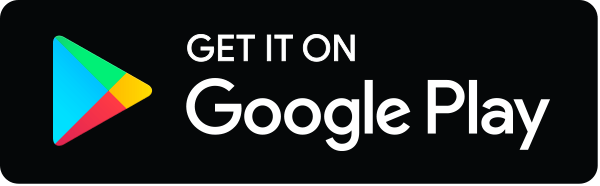