Fig. 12.1
Development process of a multimaterial, patient-specific model of aortic stenosis. Step 1 CT imaging dataset converted into DICOM format. Step 2 Segmentation process includes identification of target anatomic geometry and creation of segmentation mask. Step 3 Reconstruction of 2D segments into 3D virtual model. Step 4 Digital patient-specific model adjusted for coupling within a flow loop. Step 5 Multimaterial 3D-printed patient-specific model. Modified with permission from Vukicevic et al. [22]
Manufacturing a complex, multimaterial model is possible using PolyJet technology. This technique uses additive manufacturing, a process in which a 3D printer (Objet500 Connex 3) sprays fine layers of liquid photopolymers, 16 μm thick, along with a support material onto a platform, while a UV light instantaneously cures each layer. At the end of the 3D printing process, the support material is dissolved, leaving only the anatomic structures of interest. A schematic outline of the development process of transforming the volumetric CT data into a physical model is shown in Fig. 12.1.
PolyJet technology is capable of fabricating a wide range of AS geometries including bicuspid valves and various arrangements of calcific depositions. Morphological accuracy of replicated anatomic models is analyzed by comparing the CT scans of the AS models to the patients’ CT scan or echocardiographic images. An example of a CT scan comparison of a patient with the model created from the image dataset is shown in Fig. 12.2, while the echocardiographic images of the patient and the corresponding model are shown in Fig. 12.3a, b.



Fig. 12.2
Fabrication of patient-specific models. Short-axis images of each model are shown in the three steps of the model fabrication process. First, CT-DICOMs are converted into STL files in computer-aided design (CAD) software and then 3D-printed using two different materials simultaneously. Note the correspondence of calcific nodules (bright white and yellow) and leaflet geometry throughout the model creation process. Images adapted with permission from Maragiannis et al. [21]

Fig. 12.3
A typical flow loop setup for functional replication of aortic stenosis. Flow loop contains mock ventricle, compliance elements (c), resistance elements (R), reservoir (Res.), valves (V), pressure, and flow transducers (p and Q, respectively). a Echocardiographic image of the aortic valve in patient; b echocardiographic image of the model of aortic stenosis of the same patient; c patient Doppler interrogation of the flow through a stenotic aortic valve; and d Doppler velocity profile recorded in the patient-specific aortic stenosis model. Modified with permission from Maragiannis et al. [21]
Functional Modeling
Aortic stenosis is particularly well suited for functional replication with 3D modeling. This is because the relatively fixed valve that characterizes calcific aortic valve disease demonstrates little motion throughout the cardiac cycle. As such, the replicated aortic cusps do not need to demonstrate normal cusp motion throughout the cardiac cycle, although a subtle expansion of the stenotic valve orifice with augmented flow volume has recently been demonstrated [20]. This flow dependency of the 3D-printed valve area is consistent with the behavior of native AS cusps [20].
Maragiannis et al. [20, 21] reconstructed the first functional, multimaterial, patient-specific AS models and replicated the clinical hemodynamic conditions of aortic stenosis using an in vitro environment. The obtained models have been coupled to a custom-designed flow loop shown in Fig. 12.3, replicating the hemodynamics of aortic stenosis. The flow loop consists of compliance and resistance elements tuned to physiological values and a pulsatile mock ventricle pump that ejects the fluid through the model. Quantification of forward flow and AS stroke volume is achieved using in-line flow transducers. Peak pressure gradient across the 3D-printed valve is measured using high-fidelity pressure transducers positioned proximal and distal to the modeled valve.
The final step before 3D printing each model is to design and affix coupling segments onto the patient-specific geometry to allow incorporation of the model into the flow phantom. This typically consists of designing appropriate tubing connectors. Within the software environment, the continuity of the model surface and elimination of intersections between segmented geometries are assured [18, 21].
Experimental evaluation of patient-specific AS hemodynamics has been performed using both echocardiography and cardiac MR techniques. Doppler analysis of velocity profiles across the AS model under varied flow conditions has been reported (Fig. 12.3). Transvalvular peak and mean Doppler-derived pressure gradients were comparable to those measured in a 3D-printed model using high-fidelity pressure transducers. Flow estimates from clinical study of Doppler stroke volume were compared against flow volume as calculated using calibrated ultrasonic flowmeters positioned upstream and downstream to the 3D-printed AS model. 2D Doppler quantification of systolic flow based on continuous-wave Doppler peak and mean gradient in the 3D-printed phantoms was compared to the actual clinical data of the patient (Fig. 12.3). These studies confirmed the reproducibility of clinical hemodynamic parameters using functional 3D-printed AS models. Key hemodynamic features of the AS, including aortic valve area and peak and mean flow velocity, were accurately replicated with good agreement between clinical patient data and the 3D patient model within the flow phantom (Fig. 12.3c, d).
3D Printing Material for Aortic Stenosis
Multimaterial 3D printing allows for accurate replication of complex 3D models integrating multiple anatomic structures. Printing the regional material properties of tissue and calcific structures integrated in the same 3D model requires careful selection of materials. Patient-specific models are typically manufactured from Sylgard (Dow Corning), TangoPlus (Stratasys), or HeartPrint Flex (Materialise) materials, of which the TangoPlus material has mechanical properties most similar to select human tissue [23].
PolyJet technology represents a 3D printing technique that permits the use of a wide range of liquid photopolymers with different elastic properties, from rubber-like to rigid, transparent, translucent, or opaque, and with a wide spectrum of available colors. The choice of 3D printing material is based on the mechanical properties of the anatomic structure that is being replicated. Fused material selection is a pivotal step in the modeling process and has required preliminary definition of soft tissue and hard calcium characteristics [24, 25]. Aortic root, ascending aorta, and aortic valve cusps can be printed using a flexible material (TangoPlus, Stratasys), while the calcium is usually 3D-printed from a rigid material (VeroPlus, Stratasys). Elastic properties of rubber-like TangoPlus material are expressed by Young’s modulus or elastic modulus and calculated as stress-to-strain ratio. The print material frequently used for the noncalcified anatomic regions (TangoPlus) has a manufacturer-reported elastic modulus of 0.146 MPa at 20% strain. The print material used for the calcified anatomic region (VeroWhitePlus) has a manufacturer-reported elastic modulus of 2000–3000 MPa. In the study by Maragiannis et al., tensile testing of the replicated aortic root tissue material was 0.4 MPa, that is comparable to the biaxial testing result of human aorta root tissue reported as 1.24 ± 0.563 MPa [25]. Other recent studies showed that the flexible TangoPlus material is suitable to accurately replicate AS geometry and functional properties of aortic valve leaflets under small deformations [26].
Applications
Translating 3D imaging information into 3D digital anatomy and 3D print multimaterial, anatomically accurate models of the aortic valve complex, with all functional parts, represents a significant advancement in postprocessing of medical images. Replicating the biomechanics of aortic structures allows for highly accurate evaluation of diseased patient-specific physiology and represents a potentially very powerful new tool for assessment of acute hemodynamics that are difficult to quantify using current noninvasive imaging methods. In addition, such models facilitate accurate testing of novel medical devices and their design improvements such as those used for TAVR.
Being able to 3D print patient-specific geometry before an interventional cardiac procedure and to explore different interventional techniques could save time in the procedure room, increase efficiency of the procedure, and decrease the risk of unpredicted outcomes.
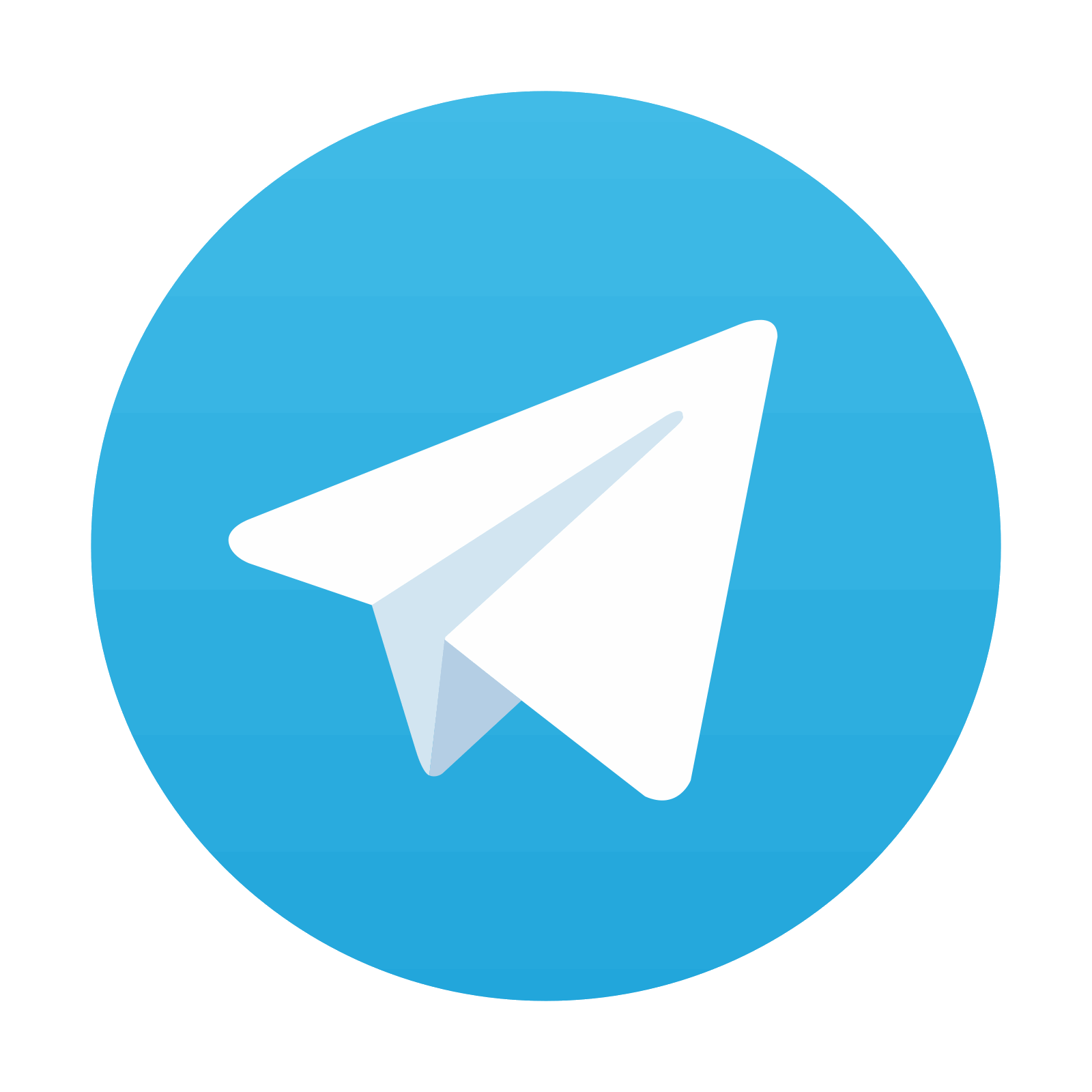
Stay updated, free articles. Join our Telegram channel
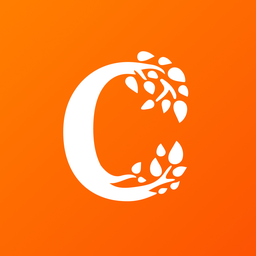
Full access? Get Clinical Tree
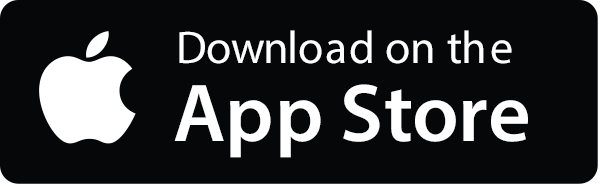
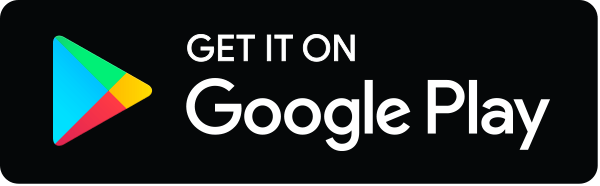