Tissue engineering is a developing science, comprising elements of engineering and biology, whose aim is to build replacement tissues de novo, from individual cellular and structural components. The impetus for our work on tissue-engineered cardiovascular structures arises from the need to replace cardiovascular tissues that failed to develop normally during embryogenesis or have become dysfunctional as a consequence of disease. In pediatric patients, the cardiovascular structures most often afflicted by congenital anomalies involve the cardiac valves and great vessels, and the theoretical advantages of a tissue-engineered structure containing live cells are the ability to grow, remodel, and repair. Although growth potential is not a consideration for the adult population, durability of valve structures remains an important issue for bioprosthetic valves, and a tissue engineering approach offers the potential to improve durability by providing the repair and remodeling capability.
Diseases of the heart valves and large “conduit” arteries account for approximately 60,000 cardiac surgical procedures each year in the United States, and all of the currently available replacement devices have significant limitations.1,2 Ideally, any valve or artery substitute would function like a normal valve or artery. For valves, this function includes allowing pulsatile blood flow without a transvalvar gradient or valvar regurgitation. The theoretical advantage of engineered tissue replacements is that they could also display other desirable characteristics, such as (1) durability, (2) growth (for infants and children), (3) compatibility with blood components and the absence of thrombosis or destructive inflammation, and (4) resistance to infection. None of the currently available devices, constructed from either synthetic or biologic materials, meet these criteria. Mechanical heart valves are very durable, but they require anticoagulation to reduce the risk of thrombosis and thromboembolism.1,2 Therapeutic anticoagulation carries associated morbidity, and even among patients who receive therapeutic anticoagulation, the incidence of thromboembolic complications of mechanical heart valve replacement is not zero.1,2 Biologic valves, whether of allograft or heterograft origin, remain subject to structural deterioration after implantation.2-4 Neither mechanical nor biologic valves have any growth potential, and this limitation represents a major source of morbidity for pediatric patients who must undergo multiple reoperations to replace valves and/or valved conduits during the period of maximum somatic growth.
Tissue engineering is an approach based upon the hypothesis that when properly designed and fabricated, these “living” devices will simulate the biology of normal cardiovascular structures, thereby overcoming the shortcomings of currently available heart valve replacements. Of particular relevance to pediatric heart surgery is the long-term function of the engineered valve over time and the potential capacity to grow, self-repair, and remodel. This chapter summarizes some of the progress that has been made in tissue engineering research as it relates to cardiac valves and conduit arteries, and then outlines the areas where additional efforts must be focused in order to direct cardiovascular tissue engineering toward clinical utility.
Heart valves open and close approximately 40 million times per year; this coordinated function occurs under the demands of hemodynamic forces of blood pressure and shear stress. The normal semilunar valve presents minimal resistance to opening and no pressure gradient during systolic forward flow. In diastole, the same structure is responsible for rapid and complete closure in order to prevent valve regurgitation. Semilunar valves must additionally resist pressure differences between the diastolic arterial pressure and the diastolic ventricular pressure. The atrioventricular valves must function in a comparable way, opening with minimal resistance during diastole and closing to prevent regurgitation during ventricular systole.
Like many other tissues, valve cusps are composed of cells residing within, the extracellular matrix (ECM). In addition, these same cells act reciprocally on the ECM to which they are attached and receive signals from the ECM. The effects of these cellular level mechanical signals on cell phenotype and behavior have been characterized by Ingber5,6 using the term “tensegrity” to describe the interactions between cell adhesion to ECM and the nucleus mediated by the cellular cytoskeleton. Semilunar valve cusps are thin, flexible structures with impressive microscopic and molecular complexity. Much of the strength and flexibility of normal heart valve cusps is due to the specialized proteins and polysaccharide-protein complexes of the ECM, produced by resident valve interstitial cells.7,8 The microscopic and molecular structure of adult valves reflects the regional mechanical forces experienced by the valve cusps; valve cusp ECM is not homogeneous, and the arrangement of the ECM provides a high degree of flexibility during systole, and a high degree of strength to resist pressure loads during diastole.7 On the surfaces, a specialized endothelium prevents thrombosis, but also acts as a transducer of mechanical force.9,10 Beneath the endothelium, valve interstitial cells receive signals sent by the endothelium, and respond to imposed mechanical signals by secreting suitable matrix.5,6,11
Semilunar valve ECM is stratified into layers and its distribution is related to valve mechanics.12 Facing the sinus of Valsalva, where eddy currents occur and diastolic pressure loads are imposed, dense collagen is found in the fibrosa layer. A middle layer of connective tissue, the spongiosa, is particularly rich in glycosaminoglycans, large complex molecules which associate with water, and this layer is thought to act as a “shock absorber,” bearing largely compressive mechanical loads. The ventricularis, an elastin-rich layer facing the lumen and the flow orifice of the semilunar valve is specialized to stretch as the cusps elongate during ejection in systole. The resulting composite structure of the native semilunar valve is anisotropic, with less elasticity in the commissure to commissure direction and greater elasticity in the annulus to free edge direction. The orientation of the collagen bundles in the native semilunar valves have also been demonstrated to affect normal leaflet motion during systole and diastole.13–15
Valve structure and function are therefore closely related, and strategies to engineer valves are aimed to mimic normal valve structure and function, potentially at the subcellular, cellular, tissue, and whole heart valve levels.
Valves do not begin development as stratified tissues; as circulatory patterns evolve in fetal and postnatal life, valve morphology also changes.12 Embryologic valve development, therefore, has relevance to tissue engineering, as a model for normal in vivo valve remodeling as programmed by gene regulatory pathways and by applied biomechanical force.
By day 15 of human embryo development, specification of myocardial and endothelial cardiac cells occurs. At day 21, formation of a linear heart tube occurs. These events are followed by rightward (dextro) looping of the heart, resulting in orientation of the cardiac chambers into their final adult positions, and opposition of two specialized segments of ECM, known as cardiac jelly.16 The first evidence of valvulogenesis occurs in this cardiac jelly, when a subset of endothelial cells separate from the luminal surface, invade the ECM, and undergo endothelial-to-mesenchymal transformation (EMT).17 In early fetal valve development, valve interstitial cells are highly proliferative, and reside in a glycosaminoglycan-rich, homogeneous microenvironment. Over the course of fetal development, cell proliferation slows, and production of ECM results in valve cusp elongation. During the late stages of fetal development (20 to 36 weeks of human gestation) and in early postnatal life, valve stratification into a trilaminar structure occurs, and organization and maturation of collagen fibers begins. At the time of birth, through a series of largely undefined molecular steps, changes in oxygenation and blood pressure distinguish the aortic from the pulmonary sides of the circulation.12 Transitional neonatal circulation has been associated with a change in phenotype from activated to quiescent interstitial cells in pulmonary, but not aortic, valve cusps.18 Valve maturation and remodeling continue during childhood; cellularity of valve interstitial cells continues to decrease into adulthood.12 Once thought to be passive structures, increasing evidence shows cardiac valves to be dynamic organs. Mechanistically, the finding that cellular and extracellular components mature is thought to reflect the dramatic changes in flow and biomechanical loading conditions from fetal to adult life.13,19 Further elucidation of the genetic regulatory events defining valve growth and maturation are likely to provide important information in designing biomimetic replacement devices.
The fundamental concept of creating a tissue-engineered cardiac valve is to develop a tissue composed of living cells which will, at some point, form and remodel their own ECM and thus provide durability and growth potential. The biological and engineering challenge lies in how to provide structural organization for these cells until they are capable of forming their own mature ECM and to provide sufficient structural support and mechanical integrity until the cells form their own ECM. Therefore, the fundamental variables involved in the creation of a living “tissue-engineered” valve involve choice or manipulation of cell phenotype, induction of appropriate ECM formation by mechanical and/or biochemical signals, and provision of structural integrity and cellular organization by the scaffold until new “native” ECM formation occurs.
Approaches to tissue-engineered heart valves (TEHV) can be divided into two paradigms, based upon the type of structural scaffold employed. One approach is to use bioresorbable scaffolds (nonwoven felts, electrospun scaffolds; knitted meshes, hydrogel-based, and combinations), and the other approach involves beginning with decellularized tissue-based scaffolds. The bioresorbable scaffold approach begins with seeding cells and culturing under appropriate biomechanical conditions (static flow, pulsatile flow) and nutrient medium. With regard to tissue mechanical properties, the goal of this approach is fabrication of an adequately strong tissue with approximately constant mechanical properties, requiring that the process of scaffold degradation occurs with a reciprocal increase in ECM production. Porosity is an important characteristic of bioresorbable scaffolds as it provides a permeable framework for cell migration, nutrient supply, and waste removal. Insufficient porosity leads to nutrient deprivation and cell death. Once a sufficiently stable tissue is formed, in vivo implantation would ideally follow, placing a newly synthesized tissue, devoid of foreign elements, in the required site. An alternative approach involves use of an acellular scaffold material which can then be populated by host tissue, either by direct in-growth or seeding from the blood stream, or some combination of these two mechanisms.20,21
This de novo approach using cell-seeded biodegradable scaffolds is the one that has been predominantly employed in our laboratory at Children’s Hospital, Boston.23-29 This approach treats components of tissue as “building blocks,” constructing valves from a variety of cell types and scaffold materials. Most in vivo studies have been carried out in the lower-pressure pulmonary circulation, which is a more tolerant system than the systemic circulation. This type of approach considers several basic questions as its foundation: (1) What cell type or combination of cell types is necessary to allow the production and maintenance of an appropriate ECM? (2) To what extent can cellular phenotype be altered, guided, or “engineered” to replicate cells found in the normal valve? (3) How can these cells be spatially organized during the development of this tissue-engineered structures until the cells in the construct produce sufficient and appropriate ECM? (4) What biochemical signals are necessary during the development of these structures to ensure proper ECM production? (5) What mechanical signals are necessary for optimal tissue development and growth? (6) Should a tissue-engineered valve construct be completely developed and have the histologic and macroscopic appearance of an adult valve prior to implantation, or can further maturation of an engineered construct occur in vivo after implantation? More recently, we have utilized acellular scaffold materials with chemical composition and fabrication processes that yield anisotropic mechanics that closely resemble those of native leaflet.22
Proponents of the decellularized tissue method base their approach on the premise that ECM characteristics and signals direct cell behavior, and that the closest structure to the normal valve scaffold is the normal valve ECM scaffold itself. Human aortic valve homografts are currently implanted without tissue-type matching, are found to become mostly acellular after several months in vivo, yet retain their mechanical properties for longer periods of time. As the exact features endowing the aortic valve with this improved, but still limited durability are unknown, it is hypothesized that these features are retained after ex vivo decellularization. These decellularized scaffolds can either seeded with cells prior to implantation, or implanted without seeded cells, in the expectation that appropriate circulating cell populations will populate the scaffolds in vivo. Without seeding of cells prior to implantation, however, some decellularized matrices are insufficiently endothelialized by circulating cells or ingrowth from adjacent tissues to resist surface thrombus formation in vivo.21
The literature of TEHV demonstrates the selection of a variety of cell types, scaffold conditions, and preconditioning regimens, and overall, methods are difficult to compare and results have been inconsistent. Systematic screening of engineered tissue elements and combinatorial approaches to building tissues have recently emerged.30,31 A complete review of past investigations is beyond the scope of this chapter; however, a summary of the fundamental elements of synthetic, biomaterial-based TEHV will be considered in detail in the following sections. In vivo outcomes of these methods will also be considered.
As in embryologic valvulogenesis, the “ideal” cell type for a TEHV would fulfill the function of both the valve interstitial and endothelial cells. Conceptually, these cells could be derived from fully differentiated cells capable of ECM synthesis, or from less committed, multipotent or pluripotent stem cells with the additional potential for differentiation into multiple cell types. Alternatively, two separate cell types could be potentially used to form a valve structure with valve endothelial cells on the surface and valve interstitial cells in the inner layers of the valve. The role of host cell ingrowth from adjacent tissues and/or seeding from the blood stream represent other alternative sources of cells.
The first TEHV experiments were performed with differentiated cells from artery or vein, including vascular smooth muscle cells, fibroblasts, and endothelial cells derived from the vasculature of immature animals.20,23-25 These cells were chosen as they were readily accessible, are derived from a cardiovascular source, and can synthesize ECM proteins. A comparison of myofibroblasts from the wall of the ascending aorta with those from segments of saphenous vein revealed that the latter cells exhibit superior collagen formation and mechanical strength when cultured on biodegradable polyurethane scaffolds.32 In our laboratory at Boston Children’s Hospital TEHV based on these differentiated cells from systemic blood vessels functioned for periods of up to 4 months in vivo.20,23 However, enhanced collagen formation may be a double-edged sword. The rapid formation of new tissue in the early culture period could give rise to an overabundance of matrix elements, leading to tissue stiffness and potential tissue contraction. Early studies with dermal fibroblasts demonstrated that valve cusps constructed from these cells developed tissue contraction, which limited the ability of valve leaflets to coapt with each other, resulting in valve regurgitation.33 In addition to production of excessive or unfavorable types of ECM, mature cells may present a problem of senescence in long-term cell cultures in vitro, which limits the ability to quickly produce sufficient numbers of cells to seed a TEHV construct. Finally, the prospect of harvesting segments of artery from an otherwise normal peripheral circulation in order to obtain cells for a TEHV represents an undesirable clinical situation, and therefore led to a search for alternative cell sources for engineered valves.
The emergence of the field of stem cell biology has the potential to change the paradigm for candidate cell types for heart valve tissue engineering. As stem cells differentiate from their embryonic state, they lose pluripotency with each subsequent step. Multiple steps of differentiation form lineages of cells. In embryonic development, differentiation down a specific lineage occurs with biochemical signaling, occurring in a specific mechanical microenvironment.34 How lineage specification occurs in the developing heart is currently an area of active research, with the goal of understanding the necessary cues to replicate differentiation down valve cell lineages. In normal development, differentiating cells are subject to a rapidly changing three-dimensional extracellular environment, and development is thought to occur by signals originating outside of the cell, from molecules originating in neighboring cells, and in the ECM. The importance of these mechanical interactions between cells and their immediate environment during embryonic development has been emphasized by Ingber.6 These reciprocal interactions between cells and their environment in developing valves, the regulatory mechanisms which induce cells to secrete and respond to components of appropriate ECM, is thought to be the underlying mechanism stratifying valve cusps into their known compartments. These mechanisms are thought to be largely driven by hemodynamics in developing valves.
There is also recent evidence that stem cells with proliferative and regenerative capacities reside in many adult tissues. These stem cells are capable of not only acting locally on the tissues in which they reside, but they may also be recruited out of the circulation and enlisted in the regeneration of diverse tissues at distant sites. A recent review details emerging evidence that bone marrow-derived endothelial, hematopoietic stem and progenitor cells can also contribute to tissue vascularization during both embryonic and postnatal life.35 Visconti and coworkers have made the intriguing observation that cardiac valve interstitial cells in mice appear to originate in the bone marrow as hematopoietic cells.36 The idea that bone marrow contains cells capable of repairing damaged tissue has been applied to regeneration of cardiac muscle after myocardial infarction, whereby progenitor cells have been isolated from sites outside the heart, then injected back into the heart in an attempt to regain contractile function of ischemic or infarcted myocytes. Results of these trials have been equivocal, marginal, or negative, suggesting that the process of regeneration does not occur by the simple addition of multipotent cells.37 The early experience with the SynerGraft decellularized heterograft valved conduits which were implanted in children as right ventricle-to-pulmonary artery conduits occurred with the expectation that circulating cells from the bloodstream, or ingrowth from adjacent normal tissue would repopulate the grafts and grow. In this instance, repopulation of the graft by circulating cells occurred, but did not result in adequate function or tissue growth.38,39 Nonetheless, the progenitor cell populations represent an attractive source of cells for tissue engineering and regeneration, because they have the plasticity necessary to fulfill critical cell functions, and are potentially programmable for lineage specification. In addition, these cells can be obtained less invasively than differentiated cells.35 Our initial experience with progenitor cells in the Mayer laboratory was gained with autologous endothelial progenitor cells (EPCs) isolated from circulating blood in lambs and seeded onto decellularized arterial segments.20 These seeded arterial grafts were then implanted as an interposition graft in the carotid artery of the donor lamb. These grafts remained patent and functional for up to 130 days. Subsequent animal studies by Sutherland and associates used bone marrow mesenchymal stem cells (MSC) to seed a bioresorbable scaffold formed into a three-leaflet valve within a conduit (Fig. 63-1). These valved conduits were implanted as valved conduits into the main pulmonary artery of neonatal sheep, and remained in place for up to eight postoperative months.27 This study was followed by that of Gottlieb et al, who implanted valves of similar elements into larger numbers of sheep and followed changes in function with growth of the animals using magnetic resonance imaging (MRI) and echocardiography. While the valves had trace to mild regurgitation at the time of implantation (Fig. 63-2), a loss of valve leaflets surface area was observed, which correlated with increasing valve regurgitation over time.29 Importantly, the valve leaflets underwent a remodeling process in vivo after implantation, seen also in earlier experiments using myofibroblasts and endothelial cells from systemic arteries, and in both sets of experiments, a layered histologic appearance developed after implantation.23,27
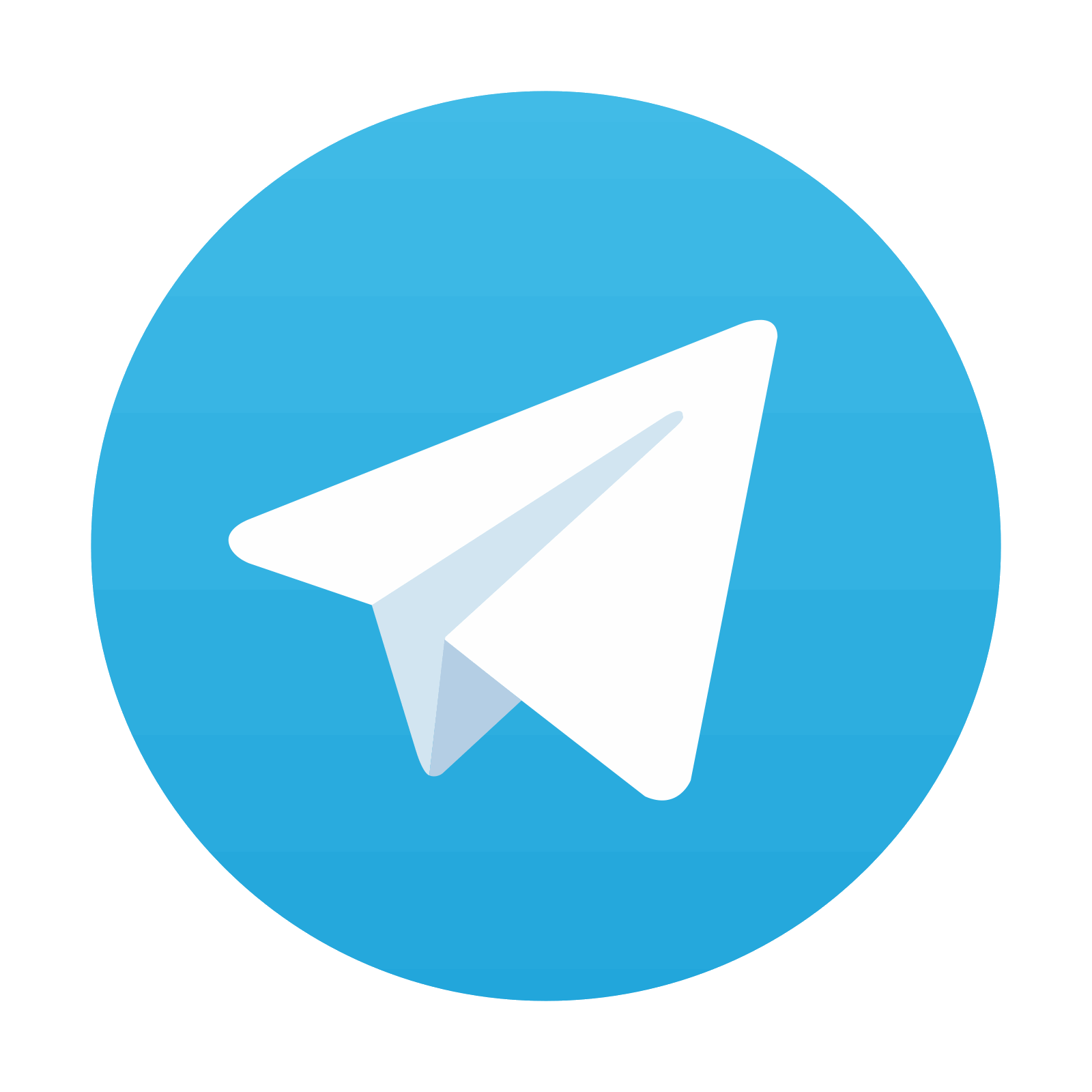
Stay updated, free articles. Join our Telegram channel
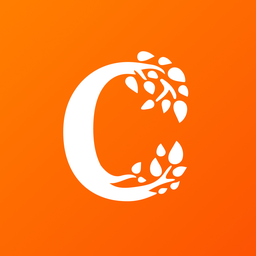
Full access? Get Clinical Tree
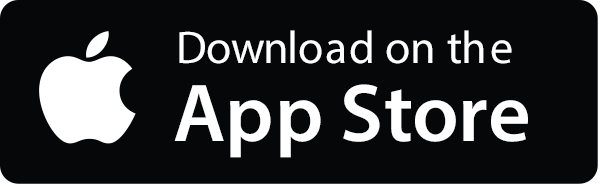
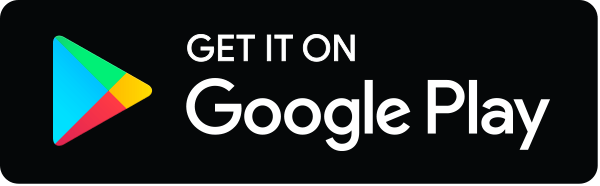