(1)
Project-team INRIA-UPMC-CNRS REO Laboratoire Jacques-Louis Lions, CNRS UMR 7598, Université Pierre et Marie Curie, Place Jussieu 4, 75252 Paris Cedex 05, France
Abstract
Tissue growth as well as repair of tissue injury is controlled by many regulators such as growth factors that both reorganize the cytoskeleton and cell adhesions and trigger the action of transcription factors, thereby enabling proper cell migration and lodging as well as adaptation to environmental conditions. Cell wall extensibility and junctional communications are involved in tissue growth. In adults, cell differentiation happens during normal cell turnover as well as during tissue repair. Tissue remodeling caused by a sustained, time-dependent mechanical stress field and chemical cues, possibly associated with angiogenesis, rely on the coordination between regulated cell proliferation, differentiation, migration, and apoptosis to determine the correct tissue configuration[1376].
Tissue growth as well as repair of tissue injury is controlled by many regulators such as growth factors that both reorganize the cytoskeleton and cell adhesions and trigger the action of transcription factors, thereby enabling proper cell migration and lodging as well as adaptation to environmental conditions. Cell wall extensibility and junctional communications are involved in tissue growth. In adults, cell differentiation happens during normal cell turnover as well as during tissue repair. Tissue remodeling caused by a sustained, time-dependent mechanical stress field and chemical cues, possibly associated with angiogenesis, rely on the coordination between regulated cell proliferation, differentiation, migration, and apoptosis to determine the correct tissue configuration [1376].
Repair, or healing, involves a series of rapid infiltrations and accumulations of various cell populations that deposit a new matrix and mature (Table 11.1). Once they have completed their tasks, some involved cell types are eliminated prior to the progression to the next phase of healing via apoptosis (Vol. 2 –Chap. 4. Cell Survival and Death). Apoptosis allows elimination of entire cell populations without tissue damage or inflammation.
Period | Events |
---|---|
Day 1 | Hemostasis |
Vasoconstriction | |
Cell influx (neutrophils) | |
Week 1 | Vasodilation; inflammation |
Cell influx (macrophages, lymphocytes, fibroblasts) | |
Growth factor signaling; cell proliferation | |
Collagen deposition | |
Month 1 | Remodeling |
Collagen crosslinking |
During the proliferation phase of tissue repair, collagen-3 prevails. During the maturation, collagen-3 is gradually degraded and collagen-1 produced. Moreover, collagen fibers that are originally disorganized rearrange, crosslink, and align along tension lines [1377]. Blood vessel density decays as local activity attenuates. Some blood vessels are removed by apoptosis.
When cells undergo apoptosis, tiny regions of the plasma membrane pinch off and form vesicles, the so-calledapoptotic bodies, that are shed and carry messages from dying cells to healthy ones to promote repair. Apoptotic bodies from dyingendothelial cells that are taken up by healthy endothelial cells heightens the expression of the Cxcl12 gene [1378].Chemokine CXCL12 counteracts apoptosis and recruits progenitor cells for repair. In apoptotic bodies,microRNA-126 prevents the synthesis of an inhibitor of CXCR4, the CXCL12 receptor. This GPCR then triggers afeedback loop that augments the production of CXCL12 to attract progenitor cells for new tissue generation.
11.1 Organogenesis
Organogenesis during embryonic development relies on differentiation and replication ofstem andprogenitor cells down to terminal differentiation and proliferation of differentiated cells. Organogenesis is achieved in sequential stages from the formation of an anlage, invagination of the primary bud, reiteration into various branches, to organization into specific proximal and distal structures.
11.1.1 Ecto-, Endo-, and Mesoderm
Organogenesis is the process by which theecto-,endo-, andmesoderm develop into organs (between week 3 and 8 of in utero life; Table 11.2). Regulators control the formation of spatial patterns as well as timing of patterning events.
Table 11.2
Organogenesis (Source: Wikipedia). Cells that migrate inward along the archenteron form the inner layer of the gastrula, which develops into the endoderm. Mesoderm gives rise to: (1) chordamesoderm (axial mesoderm) along the central axis under the neural tube; (2) paraxial mesoderm on both sides of the neural tube that generates somites (origin of vertebral column, dermis, and skeletal muscles) and branchial arches (facial muscle and cartilage, etc.); (3) intermediate mesoderm between the paraxial mesoderm that forms the urogenital system; and (4) the lateral plate (or hypomere) at the periphery of the embryo that splits into 2 layers, the somatic (body wall) and splanchnic layer (circulatory system and gut wall). The ectoderm that emerges first and forms from the outermost of the germ layers, has 3 parts: external ectoderm (surface ectoderm), neural crest, and neural tube (neuroectoderm).
Germ layer | Organ products |
---|---|
Endoderm | Respiratory tract |
Endocrine organs | |
Gastrointestinal tract | |
Mesoderm | Circulatory apparatus |
Mesenchyme (blood and lymph vessels, blood cells, | |
bone, cartilage, and connective tissues) | |
Mesothelia (pericardium, pleura, and peritoneum | |
Muscles | |
Digestive tract | |
Reproductive apparatus | |
Urinary tract | |
Ectoderm | Nervous system |
Skin | |
Nose and mouth epithelia | |
Eye |
Differential adhesion and tension as well as directed cell migration determines progenitor sorting. Differential adhesion hypothesis of organization of cell layers during the development of embryonic tissues states that in 2 cell populations that aggregate the more cohesive one (with the stronger homotypic adhesion) distributes in the middle and the less cohesive one on the outside [1379, 1380]. The spreading of one embryonic tissue over another and the formation of intertissue borders are supposed to behave like immiscible liquids. Adhesive forces between aggregated cells play the same role in cell sorting as intermolecular attractive forces in liquid surface tension. Distinct expression of cell adhesion molecules, especially cadherins, regulates histotypic sorting out of cells of embryonic tissues when cells are intermixed.
Differential surface contraction theory of morphogenesis states that cell cortical tension developed by the cytoskeleton rather than adhesion between cells generates cell sorting [1381]. In addition, signaling between mesenchymal and epithelial cells influences organ morphogenesis. Epithelial wrapping controls mesenchymal shape change and sorting of enclosed mesenchymal cells [1382].
Measurements of cell adhesiveness and cortical tension in the 3 germ layers of zebrafish embryos using atomic force microscopy show that the strength of homotypic adhesion decays from mesoderm, then endoderm, to ectoderm [1383]. The magnitude of measured cortical tension diminishes from ectoderm, then mesoderm, to endoderm.
Differential actomyosin-dependent cell-cortex tension regulated byNodal–TGFβ signaling directs progenitor sorting. Ecto- and endoderm cells occupy the cell aggregate center and periphery, respectively. However, in vivo configuration is defined by outer ectoderm and innermost endoderm, with mesoderm in the middle. Strong interactions of germ-layer cells with enveloping layer cells can invert in vitro sorting to that attained in vivo. Furthermore, groups of cells do not behave like organized sheets of cells.
11.1.2 Precursor Cells
During the early stages of embryogenesis, the fertilized egg starts to divide into blastomeres.Embryonic stem cells (ESC), derived from the totipotent cells1 of preimplantation embryos are pluripotent cells (Fig. 11.1).
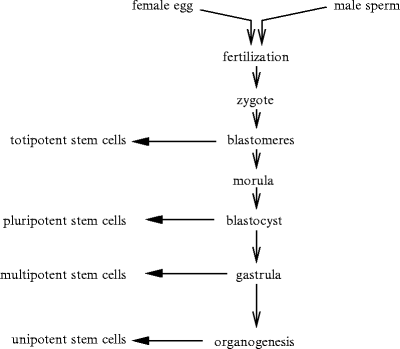
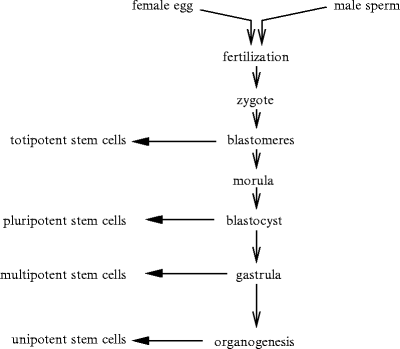
Fig. 11.1
Different types of stem cells. Fusion between female and male gametes (fertilization) leads to the zygote and embryogenesis. After fertilization, a set of fast mitoses occurs (cleavage) with formation of blastomeres building the blastula. In mammals, the blastula is called the blastocyst. The morula corresponds to an organized cell set with external and internal cells. After the mitosis rate has slowed down, the blastomeres move (gastrulation), forming the gastrula with 3 germ layers: ecto-, endo-, and mesoderm.
Pluripotent stem cells either self-renew continuously or form the various types of mature, functional cells. Pluripotent embryonic stem cells derive at various stages of embryo development according to their type, embryonic germ cells occurring at an earlier phase than others.2
Themorula (morus: mulberry; 12–32 blastomeres within the zona pellucida) corresponds to an early stage of embryonic development produced by the division of the zygote. After reaching the 16-cell stage, cells of the morula differentiate.
The central, fluid-filled blastocyst cavity (a.k.a. blastocele and cleavage or segmentation cavity) appears and the morula becomes a hollow spheric blastocyst (blastulation). The inner and outer blastomeres form the inner cell mass and trophoblast, respectively. Theblastula (blastosphere) that follows the morula consists of a spherical layer of about 128 cells surrounding the blastocele. At the blastula stage, the cell cluster contains nearly identical cells.
During the next stage, thegastrula is characterized by structured tissues. Gastrulation is the first stage in vertebrate development when different progenitor types sort out and assemble into 3 germ layers: ecto-, endo-, and mesoderm. The germ layers in tissue development involve 3 processes: folds, splits, and condensation.
After gastrulation, pluripotent embryonic stem cells give birth to multipotent stem cells that are irreversibly programmed for a given tissue. They form: (1) mesoderm, from which are derived connective tissue, muscles, and circulatory apparatus; (2) ectoderm (nervous system, skin, etc.); and (3) endoderm (respiratory apparatus, digestive tract, and endocrine glands).
Tissue and organ development includes several stages under the control of the genetic code: (1) acquisition of cell identity and transient activation of specific regulatory genes; (2) stabilization of the regulation and exclusion of alternative regulatory states byfeedback; (3) reception of signals required for development of embryonic cell lineages with respect to neighboring lineages and lineage-specific activation of organogenic genes.
11.1.3 Organogenesis, Vascularization, and Innervation
Organ development requires the differentiation and coordinated development of nerves and blood vessels that contain many cell types. The peripheral parasympathetic nervous system innervates many organs during embryogenesis.
In mouse embryos, the submandibular salivary glands appear at mid-gestation as small epithelial buds on the floor of the oral cavity. Buds and stalks of the developing submandibular gland containmultipotent stem cells. Nerves that originate fromparasympathetic ganglia innervate epithelial buds to support their development via neurotransmitter acetylcholine andacetylcholine muscarinic M1 receptor. At least in mouse embryonic salivary glands, parasympathetic innervation preferentially signals to keratin-5 + , epithelial progenitor cell population via muscarinic receptor [1385]. Furthermore, cholinergic stimulation triggers the release of heparin-binding epidermal growth factor that binds to epidermal growth factor receptor. These progenitor cells are then maintained in the adult salivary gland. Therefore, innervation not only controls organ function, but also contributes to organ repair and regeneration, as it maintains a progenitor cell population.
11.1.4 Regulation of Organogenesis
Organogenesis regulation involves various DNA-binding proteins, such as transcription factors, nuclear receptors, and their coactivators and inhibitors, as well as signaling messengers, such as growth factors and hormones, in addition to mechanical constraints. Triggered pathways modulate cell functioning and secretion of constituents of the extracellular matrix and its organization. Various growth factors and hormones bind to their specific receptors and provoke protein synthesis, and post-translational modifications of proteins, as well as exocytosis of substances. Any morphogen influences the fate of its target cells in a dose-dependent manner to direct regional specialization within a developing tissue or organ.
Many other regulators influence embryo- and fetogenesis, as they modulate gene transcription for patterning factors, such as vitamin-A and its metabolites [1386].Retinoic acid contributes to the control of cell division and differentiation in tissues of ecto-, endo-, and mesodermal origin via cognate transcription factor, the nuclear retinoic acid (RAR or NR1b) and retinoid-X (RXR or NR2b) receptors.
Retinoic acid, the active form of vitamin-A, has many functions during embryogenesis. In adults, it regulates fertility, maintains normal vision, and prevents tumor growth and degenerative diseases. This small lipophilic molecule binds to nuclear receptors that are heterodimers of retinoic acid receptors and retinoid X receptors (RAR–RXR complexes; Vol. 3 – Chap. 6. Receptors) to regulate the transcriptional activity of target genes. Retinoic acid is synthesized from inactive precursors (retinol or β-carotene) from food after digestive processing that leads to plasmatic transport and after placental transfer in embryos.3 Retinoic acid controls the activity offibroblast growth factor andsonic Hedgehog [1387].
Among transcription factors expressed predominantly in the heart, members of theGATA set — GATA4, GATA5, and GATA6 — require vitamin-A [1386]. Retinoid-regulatedcardiogenesis also involves transcription factor of the Msh homeobox class Msx1 that also needs vitamin-A input. Cardiac abnormalities result from lack or excess of retinoids.
Specification of any embryonic cell lineage is controlled by the sum of activities of transcription factors — activators and repressors — that result from interactions between involved genes that encode these transcription factors following a given signaling. Many genes indeed participate in specification and differentiation of embryonic cell lineages and progressive organization of tissues and organs. Development genes coordinate the sequence of interactions between genes that encode transcription and signaling factors.
Long and small non-coding RNAs (ncRNAs) regulate organogenesis [1388]. Small ncRNAs are associated with heterochromatin formation via the RNA interference pathway. Long ncRNAs are linked to gene clusters. Non-coding RNAs regulate transcription, as they interact with transcription factors, RNA polymerases, or DNA. Short interspersed elements of the genome transcribe non-coding RNAs that repress specific genes by binding to and inhibiting RNA polymerase-2.
The cell’s capacity to differentiate with specialized attributes is, at least partially, achieved using the genetic code. In the large majority of cases, cells acquire their fate owing to their lineage or signaling from adjoining cell lineages. In addition to the deterministic program, the differentiation pathway can be randomly fullfilled to cope with adverse changes in the environment [1389].
Cells interact with adjoining cells and the extracellular matrix owing to cell adhesion molecules such asintegrins that serve as receptors of matrix constituents. Cell adhesion molecules link intracellular cytoskeletal proteins of adjacent cells as well as cytoskeleton to matrix constituents. Matrix constituents influence intracellular events via their receptors that lodge at the cell surface, thereby modulating cell differentiation, migration, and polarization. In addition, cells release matrix-degrading enzymes and their inhibitors to remodel their nearby environment.
In kidney genesis, epithelial–mesenchymal interactions require connections ofα8β1-integrins to extracellular matrix protein nephronectin [1390]. Nephronectin and α8β1-integrin are synthesized in the ureteric bud epithelium and metanephric mesenchyme, respectively.
11.2 Cell Differentiation
During cellular differentiation, an immature cell becomes a mature specialized cell. Cell differentiation occurs not only during embryogenesis, but also in adults. In particular, adultstem cells generate differentiated daughter cells for tissue repair and during cell turnover. Cell differentiation modifies cells size, shape, polarity, metabolic activity, and responsiveness to signals due to modifications in gene expression. Dedifferentiation allows differentiated cell to revert to an earlier developmental stage.
Three basic cell classes include germ, somatic, and stem cells. Germ line cells give rise to gametes (eggs and sperm).Totipotent cells (zygote and early embryonic cells) can differentiate into all cell lineages.Pluripotent stem cells (e.g., hematopoietic and mesenchymal stem cells) are able to differentiate into many cell types.Multipotent stem cells andunipotent progenitors give rise to functional cells.
Each differentiated cell type expresses a gene subset of the genome due to a peculiar pattern of gene expression. Growth factors control the switch from one gene expression pattern to another. Distinct cell types differentially retrieve the genetic information encoded in the genome. Transcription factors andmicroRNAs form the largest families of gene regulatory factors [1391]. Sets of combinatorially expressed transcription factors and microRNAs delineate cell types. Transcription factors and microRNAs share many similar features although they have their specific properties that determine specialized regulatory niches (Table 11.3). Some microRNAs are expressed in a cell- or tissue-specific manner and contribute to cell identity. MicroRNAs can control the expression of transcriptional regulators. They also regulate alternative splicing during tissue development.
Transcription factors | MicroRNAs | |
---|---|---|
Pleiotropy | + | + |
Cooperation | Cooperative binding to | Cooperative activity |
cognate DNA sequences | ||
Cooperative recruitment | ||
of transcription cofactors | ||
Recognition of | Nucleosome coating | RNA-binding proteins; |
site accessibility | folding of target | |
and binding | mRNA sequences; | |
other microRNAs | ||
Regulation | Activation or | Repression mostly; |
repression; | fast, reversible repression; | |
slower activity | quick reactivation | |
( ± translocation) | ||
Expression | MicroRNA dependency; | Post-translational |
feedback; | regulated maturation; | |
post-translational | RNA editing | |
regulation | (adenosine-to-inosine conversion); | |
(phosphorylation) | microrna cofactor modifications |
11.3 Branching Morphogenesis
The vasculature is characterized by its branched architecture. In addition, to their own perfusion vasculature, organs can contain branched structures in a limited body region, such as thetracheobronchial tree, collecting parts of renal nephons, and glandular ducts.
Branched conduit networks connect body’s environment to organs and/or conversely. Branching augments the cummulated functional surface area (e.g., trumpet shape of the cummulated surface area of the tracheobronchial tree from large bronchi to alveolar ducts).
The respiratory tract links atmospheric air to gas exchange membrane of pulmonary alveoli. This alveolocapillary membrane is crossed by blood gas. Blood circulation then carries these gases inside the cardiovascular system that irrigates and drains body tissues. The 2 serial ventilatory and circulatory networks transport oxygen to cells and remove carbon dioxide from them.
11.3.1 Airway Morphogenesis
In mammals, air enters the organism through the nasal and oral cavities and crosses the pharynx, larynx, and trachea. The trachea branches into 2 stem bronchi that give rise to densely branched left and right bronchial trees inside lung parenchymas. The bronchial tree of the human lung contains more than 105 conducting and 107 respiratory airways [1392].
During mammalian embryogenesis, lungs develop from 2 primary buds that arise in the ventral foregut. These buds undergo extensive branching under the reciprocal control of the epithelial endoderm and surrounding mesoderm. Terminal buds may contain a population of multipotent epithelial progenitors [1393]. As airways extend, descendants of these cells give rise to progenitors of the major cell types of airways, such asciliated and secretoryClara cells. Differentiation of epithelial cells begins proximally and proceeds distally. Once airway morphogenesis is complete, the lung continues to increase in size and new epithelial cells are created. Cells with Clara cell markers can give birth to ciliated cells. In the proximal airways, columnar epithelial cells give rise tobasal cells. Basal cells can self-renew and contribute to other cell lineages. On the other hand, differentiated ciliated cells do not divide [1393].
Patterning information specifies branching time and loci during development as well as the length, shape and direction of outgrowth of each branch. Branching does not occur randomly to fill available space. This process is precisely controlled. Nevertheless, coding is simplified by repeated use of a branching mechanism, using an algorithm such as that in Mandelbrot’s fractal model [1392].
11.3.2 Airway Morphogenesis Mediators
Several morphological processes withfeedbacks control branching initiation and extension that rely on activation, competition, and interaction for tip and stalk assignment in growing branches, migration, proliferation, and survival of involved cell types [1394]. Moreover, cells implicated in lumenogenesis undergo an apicobasal polarization with an apical side building the lumen (Sect. 10.2.5).
Before birth, peripheral saccules are created and pulmonary capillaries grow in close apposition to the alveolar epithelium for gas exchange. Pulmonary immaturity and lack of pulmonary surfactant cause respiratory distress syndrome. Surfactant function relies especially onsurfactant protein-B and -C, andABCa3 transporter.
Budding and growth of the respiratory tree involve numerous growth factors, signaling proteins, and matrix components, as well as transcription factors (Sect. 11.3.4.2).
11.3.2.1 Growth Factors
In mice, lung formation begins at 9.5 days postcoitum (E9.5), when the foregut endoderm invades the splanchnic mesenchyme and undergoes dichotomous branching. Branching morphogenesis depends on mesenchymal–epithelial cell signaling using fibroblast (FGF10), hepatocyte (HGF), and transforming (TGFβ) growth factors, bone morphogenetic protein BMP4, sonic Hedgehog, and Wnt morphogen (Sect. 11.3.4.2). Factors EGF, FGF, and PDGF are involved in alveologenesis.
11.3.2.2 Fibroblast Growth Factors
Fibroblast growth factors are important regulators of the respiratory tract genesis. Fibroblast growth factor-7 and -10 diffuse from the mesenchyme and activate alternatively splice variant IIIb (of extracellular loop III) of protein Tyr kinase receptor FGFR2IIIb in the nearby epithelium and induce budding that is composed of an inner endodermal epithelium and an outer mesenchyme. The expression pattern of specific FGFs at distinct steps of bud emergence controls regional endodermal cell proliferation.
Isotype FGF10 is produced by the mesenchyme at the earliest stages of lung-bud formation with 2 primordial buds from the trachea, from which the bronchial tree arises using a process of elongation, budding, and branching. Agent FGF10 stimulates proliferation of endodermal cells in the apical region of developing buds [1395].
11.3.2.3 Epidermal Growth Factor
Epidermal growth factor, transforming growth factor-α, and amphiregulin are produced by the mesenchyme and act via the common EGFR receptor [1396].
11.3.2.4 Hepatocyte Growth Factor
Hepatocyte growth factor is produced by the mesenchyme and its receptor is confined to the epithelium [1396]. Agent HGF supports action of other growth factors in branching genesis.
11.3.2.5 Insulin-like Growth Factor
Concentrations of insulin-like growth factors IGF1 and IGF2 as well as that of their receptors do not change during gestation; IGF-binding proteins (IGFBP1–IGFBP6) are developmentally regulated [1396]. Receptor of IGF1 is necessary to avoid lung hypoplasia, but does not intervene in branching formation. Receptor of IGF2 is required for normal alveolus development.
11.3.2.6 Platelet-Derived Growth Factor
Production of platelet-derived growth factor is restricted to the epithelium, whereas its cognate receptors localize to the mesenchyme [1396]. Homodimer PDGFaa contributes to branching regulation. Protomer PDGFa is required for alveolus septation.
11.3.2.7 Transforming Growth Factor
Transforming growth factor-β1 to -3 as well as receptors TβR1 and TβR2 are involved in embryonic lungs. Agents TGFβ1 and TGFβ2 are produced by the mesenchyme and epithelium, respectively [1396]. They impede the generation of pulmonary branching via TβR2. On the other hand, TGFβ3 stimulates lung development.
11.3.2.8 Bone Morphogenetic Proteins
Bone morphogenetic proteins intervene in lung development, in particular in the proximal–distal patterning. Isotype BMP4 is highly produced by epithelial cells of bud tips and, to a lesser extent, in the surrounding mesenchyme [1396]. It precludes epithelial cell proliferation.
11.3.2.9 Guidance Cues
Members of thenetrin andsemaphorin families participate in budding and branching during lung morphogenesis. Netrin-1 and -4 are produced by epithelial cells of bud stalks. They are deposited in the surrounding basement membrane, where they preclude ectopic budding and inappropriate lateral branching [1395]. Both netrin-1 and -4 are expressed at their highest levels at non-branching proximal endoderm and stalk region of growing buds. On the other hand, they are excluded from growing bud tips, where most of the branching occurs. The spatial restriction of netrins permits efficient branching genesis. In addition, netrin-4 and, to a lesser extent, netrin-1 inhibit the formation of secondary buds induced by FGF7 or FGF10 [1395]. Semaphorin-3A is expressed by the mesenchyme that surrounds the ends of buds. It organizes the size and shape of the emerging buds.
11.3.2.10 Wnt Morphogens
Wnt morphogens signal in progenitor cells of the respiratory epithelium both during development and after injury. Activation of β-catenin leads to its translocation to the nucleus and formation of a transcriptional activation complex with eitherT-cell factor (TCF),sex-determining region Y (SRY)-box gene product Sox9, orpituitary (or Paired-like) homeobox transcription factors Pitx1 and Pitx2 to cause the transcription of target genes, such as neuroblastoma-derived MYC (NMYC), Bmp4, and Fgfr2b in epithelial cells, andMYC, PITX2, and Fgfr2c in mesenchymal cells [1397]. Once bound to the mesenchymal fibroblast growth factorFGF10,FGFR2b receptor triggers the β-catenin pathway. In the mesenchyme, mesothelial and epithelial fibroblast growth factor FGF9 connects to FGFR2c to also initiate the β-catenin pathway. β-Catenin in the mesenchyme controls the differentiation of angioblasts into endothelial cells as well as proliferation of parabronchial smooth muscle progenitors [1397].
β-Catenin reduces the expression of epithelial differentiation markers during the early pseudoglandular stage of lung development [1397]. During early lung development, β-catenin signaling triggers the proliferation of epithelial differentiation marker-negative (surfactant protein SPc − and secretoglobin ScGb1a1 − )4 cells that may be precursors of SPc + , ScGb1a1 + cells. Very early SPc − progenitor cells localize to a slightly more proximal position than SPc + cells at the distal tip of the developing lung.
In adult lungs that undergo slow turnover and possess regenerative capacity via activation of endogenous stem cell populations, SPc + , ScGb1a1 + cells at the bronchoalveolar junction represent lung epithelial progenitor cells that can give rise to both alveolar and bronchiolar cell lineages, especially bronchiolar Clara cells. β-Catenin controls the balance between progenitor expansion and epithelial differentiation. During lung epithelium regeneration, the canonical Wnt pathway is activated in niches at the bronchoalveolar duct junctions that contain bronchoalveolar stem cells [1398].
Transcription factor GATA6 synthesized in the respiratory epithelium regulates the appearance timing and amount of bronchoalveolar stem cells. Its absence causes the precocious occurrence of bronchoalveolar stem cells and lack in epithelial differentiation. It activates the synthesis of the epithelial Wnt receptor Frizzled-2 [1398]. Non-canonicalFrizzled-2 receptor precludes early epithelial canonical Wnt–β-catenin signaling. Frizzled-2 also regulates effectors of non-canonical Wnt signaling, such as G-proteins andcalcium–calmodulin-dependent protein kinase CamK2. Moreover, GATA6 is needed for proper lung epithelial regeneration and differentiation of bronchoalveolar stem cells.
11.3.2.11 P38MAPK
P38α mitogen-activated protein kinase activates transcription factors, such asCAAT/enhancer-binding protein C/EBPs and Forkhead box protein FoxA2 (a.k.a. hepatocyte nuclear factor HNF3β) that are necessary for the correct differentiation of the stem cells into alveolar type-2 and Clara cells [1399]. It controls self-renewal of the lung stem and progenitor cells, as it coordinates proliferation and differentiation signals.5 In particular, it impedes the action of mitogenic factors, especiallyepidermal growth factor receptor, hence restraining the proliferation of stem and progenitor cells.
11.3.2.12 Forkhead Box Transcription Factors
Forkhead box protein FoxA2 operates in lung maturation and differentiation ofgoblet cells;FoxJ1 in proper development ofciliated cells; andFoxF1 in formation of pulmonary capillaries.
Forkhead box factor FoxM1 does not alter lung growth, in particular epithelial proliferation and airway morphogenesis, but prevents lung maturation and causes postnatal respiratory failure, as it is required for adaptation to air breathing [1400]. It is necessary foralveolus epithelial type-1 cell differentiation and synthesis of mucin-type type-1 integral membrane glycoproteinpodoplanin (Pdpn),6aquaporin-5, andsurfactant protein-A to -D. Moreover, it is a transcriptional activator of the genes that encode surfactant protein-A and -B. In adddition,FoxP2 contributes to postnatal lung alveolarization.
11.3.2.13 Other Transcription Factors
Many other transcription factors are produced in cells of the primitive lung, such asNKx2-1,GATA5 and GATA6,members of the C/EBP family,HoxA5 and HoxB3 to HoxB5, andnMyC, as well as intracellular (nuclear) receptors such as the glucocorticoid receptor (GR or NR3c1) [1396].
11.3.3 Mechanical Basis of Branching
Mechanical forces contribute to tissue morphogenesis, as they can shape epithelial sheets. Cadherins engaged in homophilic complexes between apposed cells interact with cortical actin–myosin filaments. Intercellular adhesion sites transmit forces generated in- and outside involved epi- or endothelial cells. Moreover, cadherin-based adhesion loci create forces that tend to lengthen these cell contacts, whereas cortical tensions exerted by actin–myosin filaments tend to shorten them [1394].
Monolayers of epi(endo)thelial cells that organize to locally minimize its potential energy and reach a stable equilibrium have been modeled as sheets. Mechanical modeling will then have to be applied to more complex structures, such as capillaries covered by a single cell layer and conduits coated by pseudo- and stratified epithelia, as well as epithelia associated with a mucous layer.
11.3.4 Molecular Basis of Branching
11.3.4.1 Signaling in the Vasculature
In the vasculature,vascular endothelial growth factor, especially VEGFa via VEGFR2, causes angiogenic sprouting. Tip cells emit numerous filopodia and migrate in a given direction under the guidance of VEGFa (Chap. 10). Whereas tip cell migration depends on a gradient of VEGFa, the followers — stalk cells — proliferate under the control of VEGFa concentration [1394]. Selection of tip from stalk cells results from Delta-like ligand DLL4 and its receptorNotch-1. Ligand DLL4 is preferentially produced in tip cells that reside close to a high VEGF concentration. Endothelial cells at tips of new tubes compete for leader positions. They can suppress the Notch-restricted, tip-cell phenotype of their immediate neighbors when they synthesize DLL4 and present DLL4 to adjacent migrating cells [1394]. Many other primary messengers participate in blood vessel branching such as guidance molecules (netrins, semaphorins, and slits).
11.3.4.2 Signaling in the Tracheobronchial Tree
In developing lungs, branching is bounded by a volume of mesenchymal tissue. The bronchial tree indeed develops by branching of the airway epithelium into a surrounding mesenchyme. Reciprocalfeedbacks between the airway epithelium and surrounding mesenchyme control the normal sequence and three-dimensional pattern of branching events [1392].
The branched tree can be generated by a genetically tractable, hierarchical, and modular program that contains 3 local modes of branching used in 3 different orders, i.e., at different times: domain branching at the branching onset and planar and orthogonal bifurcations [1392]. These branching modes may arise from the combination of a few elements, i.e., a master branch generator and 3 genetically encoded slaves that govern the pattern of branching events [1392]: (1) a domain specifier; (2) a genetically programmed periodicity generator that determines when side branches arise; (3) a bifurcator that defines branching loci, i.e., their occurrence time; and (4) a rotator that directs the bifurcation angle w.r.t. the stem plane. These branching modules are characterized by their coupling and repetitive occurrence.
A primary conduit constructs branching domains that serve as scaffolds. Branching arises at different positions directed by the periodicity generator to give rise to 2 coplanar branches, one of which undergoes domain branching and the other bifurcates again in a planar manner. Planar bifurcation, i.e., splitting of a branch tip into 2 branches, generates most ternary and some quaternary branches. Orthogonal bifurcation involves planar bifurcation with rotation of the bifurcation plane to give rise to higher-order generations.
Several types of sequences arise according to branching modes [1392]. In sequence 1, a founder branch formed by domain branching uses immediately and permanently the orthogonal branching mode. In sequence 2, a founder branch formed by domain branching utilizes domain branching for some daughter tubes that then employ permanently the orthogonal branching mode. The founder forms other daughter ducts by planar bifurcation that themselves undergo planar bifurcation at their tips to form domain branches along their length. These domain branches switch permanently to orthogonal bifurcation. Other sequences can be observed.
However, branching pattern can vary [1392]. Branches can originate off an unusual parent branch. A branch can miss and daughter branches spring directly from the grandparent conduit. In addition, branching network size and architecture differ among species.
11.3.4.3 Domain Branching
In mice, branches form in 4 domains: lateral, dorsal, medial, and ventral [1392]. The first secondary branch from the left primary bronchus lineage buds off the lateral sprout of the founder branch. Over the next 2 days, additional branches sprout distal to the first secondary branch and create a row of lateral secondary branches. Then, another row begins to form along the dorsal surface of the left primary bronchus. As this domain develops, a third row starts to sprout from the medial surface of the left primary bronchus. Afterward, a fourth domain eventually arises from the ventral surface. This ventral domain often consists of a single distal branch. Secondary branches off the distal portion of the right primary branch also arise by domain branching, beginning with a row of lateral branches. The branch spacing in each row is similar to the left pattern, but neither the row-initiation positions nor branch number in each row are the same.7
In domain branching, the main secondary daughter branches form in domains at different positions around the wall circumference of the parent branch, like rows of bristles on a bottle brush [1392]. However, each row is characterized by an independently patterned domain. A patterning controller of domain branching that directs the branching situation (either proximal or distal) is constituted of a periodicity generator that regulates the sequence of branching within each domain. A circumferential patterning controller specifies the positions of domains and the order with which domains are used.
11.3.4.4 Planar and Orthogonal Bifurcations
Many ternary branches are constructed by domain branching. Nonetheless, some ternary and higher-order generations arise from a different mode of tip expansion and bifurcation. Planar bifurcation corresponds to branching with similar orientation w.r.t. the founder branch, such as that of certain ternary and quaternary branches [1392].
On the other hand, some ternary and most higher-order generations use a third branching mode, the orthogonal bifurcation. Branches bifurcate as in planar bifurcation, but between each round of branching with an approximately 90-degree rotation of the bifurcation plane. The daughter tubes are thus arranged in a rosette [1392].
11.3.4.5 Genetic Control and Epithelial–Mesenchymal Feedback Loops
Lung development relies on epithelial–mesenchymalfeedback loops that usegremlin-1,bone morphogenetic protein BMP4,fibroblast growth factor, as well assonic Hedgehog andWnt morphogens, andretinoic acid. Many transcription factors, such as zinc finger protein, multitype ZFPM2,8GATA4, andNKx2.1, are implicated in pulmonary branching [1394]. Components of the extracellular matrix, such as fibronectin and laminin, are also involved [1401].
11.3.4.6 FGF
Fibroblast growth factor secreted from mesenchymal cells near to epithelial tip cells, especially FGF10 that targets predominantlyFGFR2b receptor on emerging branch epithelial cells, promotes epithelial budding, outgrowth, and branching. Signaling launched by FGF10 provokes localsprouty-2 production at the branch tips [1392]. Sprouty-2 hinders themitogen-activated protein kinase modules and limits the proliferation and migration of tip cells, hence prohibiting further branch extension, but initiating new branching. Sprouty-2 can then be associated with the periodicity generator [1392]. An elevated activity of the periodicity generator reduces interbranch length and provokes a shift from bifurcation to trifurcation.
The signaling pathways mediated by hypoxia-inducible factor and vascular endothelial growth factor cooperate with the FGF–FGFR–sprouty-2 pathway to match the developing capillary network to the growing airway tree, thus optimizing gas-exchange capacity of lungs [1401]. Moreover, FGF upregulates expression of the Bmp2 and Bmp4 genes in tip cells. Agent FGF10 may also act as a chemoattractant [1394].
11.3.4.7 BMP
Bone morphogenetic protein BMP4 as well as sonic Hedgehog signaling by tip cells exert an autocrine regulation that restrict FGF-mediated branching. On the other hand, BMP4 synthesized by mesenchymal cells enhances branching (paracrine regulation) [1394]. Factor BMP4 favors proliferation and survival of lung epithelial tip cells.
11.3.4.8 Sonic Hedgehog
Sonic Hedgehog regulates the progression rather than initiation of airway branching [1394]. It inhibits near-tip mesenchymal FGF10 synthesis, hence new branching in non-allocated loci. Therefore, sonic Hedgehog contributes to the structural reproducibility.
11.3.4.9 Wnt
Wnt morphogen uses its canonical pathway to reinforce FGFR2b expression in epithelial cells [1394]. On the other hand, non-canonical Wnt5 signaling precludes FGF10 synthesis.
11.4 Therapeutic Repair
Cell transplantation, gene delivery, and molecule administration are studied to treat various disorders. In addition, therapeutic strategies are developed either to promote revascularization of ischemic tissues and regenerate organs or inhibit angiogenesis in some diseases.
Certain organs and tissues, such as liver and skin, regenerate, but most organs lack regenerative capacity, hence being unable to repair aging, diseased, and injured tissues. Heart has a limited intrinsic regenerative capacity. Somatic cells normally cannot regenerate, except when adequately treated. Tissue stem cells generally have limited capacity.
Various types of differentiated cell can be generated from embryonic (ESC) orinduced pluripotent stem (iPSC) cells. Moreover, these cell types can be used to model diseases and optimize therapies. Regenerative medicine aims not only at stopping progression of degenerative diseases of more or less known pathogenesis, but also at reversing their course.
In addition to therapies based on stem cells and virus-injected genes, some strategies rely on locally prolonged administration of growth factors. Intramyocardial injection of self-assembling peptide nanofibers combined with VEGF can create a microenvironment that can recruit myofibroblasts and cardiomyocyte-like cells [1402]. Sustained VEGF release (14 d) improves postinfarct neovascularization in rats.
11.4.1 Stem Cells and Regenerative Therapy
Tissue repair and regeneration involve cell interaction over a long time scale to produce correct cell type, number, location, and function. A successful cell-based therapy relies on long-term stem cell engraftment and survival as well as cooperation between transplanted and host cells (Table 11.4). Performance of cell therapy indeed relies on paracrine signaling launched by transplanted cells to support stem cell homing, cell survival, angiogenesis, tissue development, and organ functioning.
Table 11.4
In the heart, transplantation of stem cells (including epicardial progenitor cells) is aimed at repairing an infarcted myocardial region upon trilineage differentiation (cardiac and smooth myocytes and endothelial cells) to recover the cardiac function, especially contractility, and myocardial blood flow (Source: [1404]). Implanted stem cells can stimulate the recruitment, survival, and differentiation of host stem cells. The former can liberate growth factors (e.g., angiopoietin-1 and insulin-like [IGF1] and vascular endothelial [VEGF] growth factors), chemokines (e.g., CXCL12), and antifibrotic mediators. Timing, delivery method (intravenous, transarterial, as well as intracoronary, transvenous injection into coronary veins, and intramyocardial, either epicardial or catheter-based transendocardial), and amount contribute to the clinical outcome.
Cell differentiation | Paracrine signaling |
---|---|
and migration | |
Tissue regeneration | Protection against apoptosis |
Adequate scar | Homing of exogenous stem cells |
Angiogenesis | Migration of endogenous stem cells |
Restitution of stem cell niches |
Regeneration potential relies on tissue-residentstem cells. However, the number of stem cells in tissues is less than 1 for 104 resident cells. Multiple stem cell populations have been discovered in various organs in adults (e.g., blood, brain, myocardium, skeletal muscle, and skin). In particular,hematopoietic stem cells (HSC) can proliferate and differentiate to produce lymphoid and myeloid cell types.Bone marrow-derived stem cells (bmSC) can differentiate into variousunipotent progenitors and then specialized cell types (e.g., cardiomyocytes).
Cells of certain tissues, such as skin, blood, and gut epithelia, have a great turnover in normal conditions and are permanently replaced. These tissues can thus be restored to their original state after injury. On the other hand, other tissues such as the central nervous system can be repaired, but not completely restored.
Stem cells modulate tissue formation, maintenance, and repair. They most often stay in relative quiescence, and reside in specialized microenvironments (niches). Stem cells both: (1) self-renew and generate additional stem cells and (2) differentiate into various progenitor cells.9
Stem cell can thus be used in therapies that require repair, replacement, and regeneration to stop and reverse damages caused by degenerative diseases or by perfusion defects such as heart failure arising from ischemic and non-ischemic cardiomyopathies.
Pluripotent stem cells such asembryonic stem cells (ESC) can differentiate into all cell types. Ethical, legal, and biological issues limit their use.
Reprogrammation of adult somatic cells into induced pluripotent stem cells or induced specialized cells such as cardiomyocytes yields an alternative. However, further investigations are required. Adult stem cells are most often only able to form their tissue of origin. In any case, therapy benefits depend on an efficient engraftment of administered stem cells, proper differentiation, and interaction with host cells.
Adult multipotent precursors offer alternatives to embryonic stem cells if they have the same potential. Stem cell function can be enhanced by suitable drugs and growth factors that promote cell growth, differentiation, survival, and homing.
Bone marrow accomodates various types of stem and precursor cells. They yield a pro-angiogenic microenvironment. Bone marrow-derived mesenchymal stem cells (bmMSC) can differentiate into multinucleated myotubes and exhibit cardiomyocyte-specific gene expression [1404].
Umbilical cord blood contains a wide variety of stem cells, such as hematopoietic, mesenchymal, and unrestricted somatic stem cells, that can generate numerous cell types.
Reprogramming of fibroblasts, B lymphocytes, hepatocytes, and gastric epithelial cells to inducedpluripotent cells was carried out using retrovirus-mediated transduction of a defined set of transcription factors in mice (octamer-binding transcription factor Oct4,SRY-related HMG box Sox2,Krüppel-like factor KLF4, andMyC). Functionally redundant KLF2 and KLF5 can replace KLF4 in fibroblast reprogramming [1405]. In addition,nuclear receptor ERRβ (NR3b2) that forms a complex withNanog and Oct4 and targets many genes involved in self-renewal and pluripotency in stem cells participates with Oct4 and Sox2 to conversion of mouse embryonic fibroblasts to induced pluripotent cells. Nuclear receptor NR3b2 can replace KLF4 in the presence of Oct4 and Sox2 and in the absence of MyC [1405].
Embryonic or induced pluripotent stem cells are most often suitable to generate given cell lineages, but have little or no capacity to commit into other cell lineages. Multipotent cardiovascular progenitors can enter cardiovascular cell lineages to give birth to distinct vascular cells. They must not only differentiate into multiple cell types (arterial, capillary, and venous endothelial and smooth muscle cells, nodal cells, and atrial and ventricular myocytes) according to types of stimulated pathways, but also keep the adequate proportion of involved cell types as well as tissue architecture. Cardiovascular master stem cell is a common multipotent progenitor that is characterized by cardiac signature gene NKx2-5.
Growth factor-mobilized peripheral blood mononuclear cells represent a suitable source of autologous progenitor cells when proper differentiation can be achieved. Activators of early cardiac lineage-restricted gene NKx2-5 include small sulfonyl hydrazones that can trigger expression of cardiac markers (e.g.,transcription factor NKx2-5,myocardin,troponin-I, and sarcomericα-tropomyosin)10 in various embryonic and adult stem and progenitor cells such as mobilized peripheral blood mononuclear cells [1406].
Except left ventriculomyocytes, most cardiac cells (vascular smooth and cardiac myocytes and nodal and endothelial cells) are derived from multipotent progenitors that express gene Islet-1 (Isl1) [1407]. A small quantity of cells that express stem cell markers such asstem cell factor receptor (SCFR), stem cell antigen SCA1, and multidrug resistance protein MDR1, have been identified in the human heart [1408]. However, SCFR + cells can be scouting cells of the immune system.
11.4.1.1 Cardiac Stem Cells
Cardiac stem cells divide either symmetrically or asymmetrically.11 Myocardium contains stem cell niches [1409]. Cardiac stem cells are smaller then mature cardiomyocytes. Cardiac stem cells and supporting cells are connected by gap and adherens junctions. Integrins of primitive and committed cells within the niche attach these cells to extracellular matrix proteins (fibronectin and laminin) that transduce mechanical signals for differentiation regulation.
Stem cells may regenerate the myocardium, especially after myocardial infarction and in ischemic cardiomyopathy, because the heart is a weakly regenerative organ. Progenitor cells secrete paracrine factors and may contribute to vasculogenesis and tissue repair and remodeling [1410].
In the heart, resident stem cells can lead to endothelial and smooth muscle cells as well as cardiomyocytes [1411]. The heart may contain various populations of resident myocardial progenitors with different expression modes that may differentiate into cardiomyocytes and endothelial cells.12
Although cardiogenic progenitor cells maintain myocardial turnover, they do not lead to heart regeneration. Consequently, injured adult hearts form scars without cardiomyocyte proliferation. Appropriate differentiation of stem cell and induction of the division cycle of resident cardiomyocytes can be stimulated with suitable factors. Cytokine treatment (e.g., colony-stimulating factor CSF3) improves infarction repair, but can also increase mortality [1412]. Cell delivery can be done either by intracoronary infusion or direct intramyocardial injection during grafting or using endovascular procedures. Periostin activates plasmalemmal αV-, α1-, α3-, and α5-integrins, and induces re-entry of cardiomyocytes into the cell cycle [1413].
11.4.1.2 Endothelial Progenitor Cells
Circulating endothelial progenitor cells (EPC) promote neovascularization; hence, they are also calledcirculating angiogenic cells. They are reduced in number. They can become dysfunctional in chronic diseases. Circulating progenitors contribute only sligthly to cardiomyocyte repopulation. Endothelial progenitors can induce myogenesis in vitro, and angiogenesis for O2 supply. A time window exists for optimal cell therapy. Infusion of EPCs promotes neovascularization after ischemia. Peptidasecathepsin-L expressed in EPCs (but not strongly in endothelial cells) degrades the matrix, thereby allowing invasion by EPCs [1414].
11.4.1.3 Skeletal Myoblasts
Skeletal myoblasts are quiescent stem cells with proliferative capacity in vitro and ischemic tolerance in vivo. Moreover, the risk of aberrant differentiation is limited [1415]. Unfortunately, implanted myoblasts do not necessarily differentiate into excitable cardiomyocytes. In particular, they are unable to couple electromechanically with cardiomyocytes. Skeletal myocytes actually do not express adhesion and junction proteins required for electromechanical coupling.
11.4.1.4 Bone Marrow Cells and Hematopoietic Stem Cells
Transplantation of bone marrow cells possibly enriched with hematopoietic stem cells can generate coronary endothelium to a greater extent than myocardium [1412]. Bone marrow cells also enhance infarct healing. Works on bone marrow-derived mononuclear cells fail to demonstrate efficiency.
Adult bone marrow cells retain dedifferentiation capacity. They can enter into cardiomyocyte and vascular lineages. Bone marrow cells engraft, survive, and grow within the myocardium and form junctional complexes based on connexin-43 and N-cadherin with resident cardiomyocytes [1416]. Implantedhematopoietic stem cells differentiate into blood cells, but not significantly into cardiomyocytes.
11.4.1.5 Mesenchymal Stem Cells
Mesenchymal stem cells (MSC) of the stromal region (non-hematopoietic compartment) of the bone marrow produce growth factors and cytokines. They colonize sites of injury. Implanted MSCs decrease pathological wall remodeling. Bone marrow mesenchymal stem cells can differentiate into cardiomyocytes and vascular cells [1417], but they can have limited survival after transplantation in the myocardium [1418]. Furthermore, cell therapy may be ineffective or even hazardous in certain clinical settings and specific subgroups of patients [1419, 1420].
Bone marrow-derived mesenchymal stem cells that overexpress the Pkb1 gene (that encode PKB1 kinase) are better for cell therapy of acute myocardial infarction. Secretion of paracrine cytoprotective factors from stem cells exerts an effect on cardiomyocytes exposed to hypoxia [1421].
A mixed ester of hyaluronan with butyric and retinoic acid acts as a cardio- and vasculogenic agent in humanmesenchymal stem cells isolated particularly from fetal membranes of term placenta. Hyaluronan with butyric and retinoic acids primes stem cell differentiation into endothelial and cardiac cells [1422].
11.4.1.6 Embryonic Stem Cells
Embryonic stem cells differentiate into all cell types, especially cardiomyocytes organized in myofibers when stimulated by suitable cardiac promoters, but they can produce teratomas. Moreover, they can form ectopic pacemakers.
Human embryonic stem cells can differentiate to cardiomyocytes using activin-A and BMP4 factors. However, a mixed cell population with cardiomyocytes, endothelial cells, and fibroblasts must be cultured in a rotating orbital shaker to create human, functional, prevascularized, cardiac tissue patches [1423]. Preformed microvessels can anastomose with the host coronary circulation.
In summary, transdifferentiation has stimulated multiple clinical trials. Cardiac progenitors from the cardiac population and cardiomyogenic cells derived from adult bone marrow stromal cells are able to differentiate into cardiomyocytes when interacting with cardiomyocytes. Regenerative cardiology trials most often use bone marrow cells and mesenchymal stem cells, because cardiac stem cells do not generate significant repair. However, the results of stem cell injection in hearts after heart attack at most show slight, transient benefit. Cell treatment optimization hence remains challenging, taking into account disease type, patient history, associated therapy for the selection of the cell type, associated growth factors, delivery route, and administration timing.
11.4.2 Gene Therapy
Genes encode transcripts for the synthesis of proteins required for cell structures and functions. Gene therapy is the delivery of genetic materials into cells using viral and non-viral gene carriers, or vectors. Gene transfer technology is aimed at correcting defective genes responsible for lesions and thus repairing diseased tissues. Normal genes are inserted within the genome to replace abnormal genes.
Current vectors are genetically modified, safe viruses (e.g., adenoviruses, lentiviruses, retroviruses, and adeno-associated virus)13 that unload the therapeutic human gene into target cells. Gene therapy vectors using adeno-associated virus can lodge without incorporating the genome (extrachromosomal residence) of host cells. In particular, recombinant human adenoviruses and adeno-associated virus efficiently deliver and express their genomes in both dividing and quiescent cells. Adeno-associated virus can cause a long-lasting gene expression with respect to adenovirus, because of its minimal immunogenicity. Major drawbacks comprise oncogenic transformation and inflammation with elimination of infected cells.
Non-viral gene transfer uses plasmids, polymers, and liposomes,14 which are less expensive and safer than viral vectors, but less efficient. Manufactured non-viral vectors with low toxicity and immunogenicity can efficiently enter into cells, but yield low gene expression.
Genetic transfer to restore a normal cardiac function struggles against cardiomyocyte apoptosis and targets angiogenesis using growth factors and cytokines (VEGFa121, VEGFa165, VEGFc, FGF4, IGF1, CSF3, and erythropoietin),15 nervous control16 (e.g., C-terminus of β-adrenergic receptors and adenylate cyclase AC6),17 and Ca2 + handling during excitation-contraction coupling (systole) and relaxation (diastole; i.e., (sarco(endo)plasmic reticulum Ca2 + ATPase SERCA2a, phospholamban, and S100 Ca2 + -binding protein S100a1) [1424].18
A combination of 3 transcription factors,GATA4,myocyte enhancer factor MEF2c, andT-box factor Tbx5, reprograms fibroblasts into functional cardiomyocytes in vitro. Transfer of the Tbx5 gene using viral vector can convert cardiac fibroblasts from the infarct region into cardiomyocyte-like cells [1426].
Sonic Hedgehog19 (Shh; Vol. 3 – Chap. 10. Morphogen Receptors) that regulates heart development during embryogenesis, can preserve, after intramyocardial gene transfer in adult animal hearts, the left ventricular function after both acute and chronic myocardial ischemia by enhanced neovascularization, as well as reduced fibrosis and apoptosis [1427].
11.4.3 Tissue Engineering
Tissue engineering20 is the construction of living tissue. Bioreactors are devices used for the growth of tissues in an artificial environment that mimicks the physiological conditions.
In vivo biomechanical21 and chemicophysical conditions that mimic the natural environment (mechanical loading such as flow in particular) are created for in vitro cell conditioning and construction of blood vessel, heart valves, etc., with features similar to the native tissue ones. Mechanical loading with a conditioning protocol (cycle frequency and magnitude range) of tissue in formation in bioreactors increases synthesis of extracellular matrix constituents much more than in the absence of dynamical loading.
Smooth myocytes cultured in a three-dimensional collagen-1 matrix, stimulated by PDGF and TGFβ and cyclic mechanical loading respond accordingly to the combination of chemical and mechanical stimuli [1428]. The produced effects depend on the nature of the extracellular matrix.
In vitro investigations on cultured cells can simplify the reality because important, more or less unknown, factors can be neglected, in particular blood supply. Signaling associated with local and regional needs as well as the state of the whole organism is neglected.
By analogy with ecology, histological niches better define the cell status, living site, functioning, and interactions with the environment. Bioreactors must then replicate the niche. For example, endothelial cells constitute a niche component of adult neural stem cells.22 Osteoblasts lining the inner bone surface are associated with hematopoietic stem cells.23
A scaffold made of natural and synthetic materials that acts as a temporary extracellular matrix, with a nourishing supply bathed with growth factors is seeded by cells that thrive and adapt to produce engineered tissue to be implanted in the body. Synthetic biomaterials are thus developed for use as three-dimensional extracellular environments that mimic the regulatory characteristics of natural extracellular matrix and ECM-bound growth factors. Support biomaterials include self-assembling fibrillar networks and other protein polymers that present bioactive ligands and respond to cell signals. Such cues can lead to remodeling and developmental processes involved in tissue-specific differentiation with suitable structure–function relationships. Besides, bone marrow cultured in growth matrix can be used to yield angiogenesis. Moreover, mesoscale tissular modules can be assembled and associated with vascular supplies to form vascularized engineered tissues with significant viable cell densities [1431].
Tissue production depends on interactions between the growing tissue and its environment. The dynamic environment within bioreactors, in particular stimulation by mechanical stresses under control to avoid cell damage significantly affect tissue growth and development.
11.4.3.1 Heart Tissue Engineering
Engineered heart tissue can form a thick myocardium after implantation on myocardial infarcts in immune-suppressed rats [1432]. When evaluated 28 days later, engineered heart tissue shows neither undelayed electrical coupling to the native myocardium, arrhythmia, nor dilation. Cardiomyocytes seeded onto mechanically conditioned, perfused, porous scaffolds can conduct action potentials and beat synchronously, but the scar hinders a proper integration of implanted cells.
Cell sheet technology applied to a monolayer of adipose tissue-derived, pluripotent,mesenchymal (non-hematopoietic) stem cells allows the repair of scarred myocardium after myocardial infarction in rat hearts [1433]. Engrafted mesenchymal stem cells prevent wall thinning in the scar area and improve the cardiac function.
Composite matrices can be used for heart valve engineering. Enzymatically decellularized porcine aortic valves that are impregnated with biodegradable polymer by a stepwise exchange provide a scaffold for heart valve tissue engineering with complete endothelialization [1434]. Ovine cell seeding of decellularized porcine heart valves (with almost complete preservation of the extracellular matrix) is improved under pulsatile flow conditions [1435].
Preliminary numerical experiments have been carried out to study the content and orientation of collagen fibers24 of the loaded aortic valve [1437]. In an isotropic cusp, the fiber orientation is driven by the principal strain directions. The remodeling depends on the transvalvular pressure, fiber stiffness, initial fiber direction (close to the principal strain directions in this work), and loading conditions.
11.4.3.2 Vascular Tissue Engineering
Sheet-based tissue engineering, using fibroblasts and endothelial cells, without smooth myocytes and exogenous biomaterials, can form cylindrical blood vessels, characterized by a composite multilayered wall with vasa vasorum [1438].
Arterial stenoses and lumen blockage by clots locally or emboli remotely can be treated by bypass grafting surgery. Autologous grafts (internal mammary artery, saphenous vein) are used in most patients. Synthetic grafts can also be utilized. To reduce the failure rate of synthetic (polytetrafluoroethylene) grafts due to thrombosis and scar within graft lumen, biocompatible, durable biosynthetic grafts have been made. Synthetic tubes are coated by an adhesion matrix (fibronectin) and endothelial cells on its interior surface. Endothelial cells are genetically changed to overexpressfibulin-5 in order to improve their adhesion on matrix and avoid flow-induced detachment [1439, 1440]. Because of their decayed capacity of proliferation, these modified endothelial cells are also genetically altered to overexpress vascular endothelial growth factor. A coating of adhesion matrix on the tube exterior surface allows seeding of smooth myocytes, which are also genetically altered to overexpress adhesion factors and growth factors.
Mesenchymal stem cells can also be used for the construction of vascular grafts. Strain influences the differentiation (particularly into smooth myocytes) and proliferation of mesenchymal stem cells, mechanotransduction depending on the orientation of cells with respect to the strain axis [1441].25 The implicated signaling pathway can be affected by the activity of the cytoskeleton and focal adhesions, both subjected to applied loading.
A better understanding of mechanotransduction processes can improve the tissue engineering setup. Numerical simulations, once validated, are important because they: (1) provide the fields of imposed stresses and (2) can optimize the bioreactor design.
11.4.3.3 Lung Tissue Engineering
Lung design is characterized by an efficient structure–function relationship. The pulmonary architecture enables ventilation, perfusion, matching of these 2 functions, and gas exchange across an optimal gas transfer surface area. The anticoagulant and -immunogenic air–blood interface is immersed in a deformable pump. Because lung tissue has a limited regeneration capacity in vivo, lung transplantation is used in some circumstances, but is limited by a shortage of donor organs. In the case of bone marrow, successful transplantation relies on tissue devitalization followed by reintroduction of a regenerating cell population. After complete decellularization of isolated adult rat lungs, the acellular extracellular matrix that preserves the hierarchical branching structure of its vasculature and airways, i.e., that keeps scaffolds of vasculature, airways, and alveoli, is reseeded with lung epithelial and endothelial cells of neonatal lungs and cultured in a bioreactor that retains the developmental environment of fetal lung [1442, 1443]. The resulting tissue contains alveoli, microvessels, and small airways with the appropriate cell types and rheology. After sevarl days, the construct can be perfused with blood and ventilated with physiological pressures.
11.5 Inflammation
The immune system responds to aggression byinflammation, a defensive event cascade. Inflammation includes: (1) effector and cell delivery to the involved region; (2) limitation of the damage spreading by a physical barrier; and (3) angiogenesis and tissue repair.
The major inflammatory events comprise: (1) an immediate local increase in blood supply with vasodilation upstream and vasoconstriction downstream from the affected region; (2) a decrease in blood velocity for cell adhesion; (3) an increase in local capillary permeability, resulting in plasma leakage in affected tissues and creating interstitial space congestion (the exudated fluid has a higher protein content than the fluid that is normally filtrated by the capillary wall); (4) an increase in expression ofendothelial adhesion molecules26 for cell adhesion and extravasation; and (5) an influx of phagocytic cells into damaged tissues.27 Later,lymphocytes enter the inflammation site.
Leukocyte chemotaxis under molecular guidance is controlled by external and internal signals that activate signal transduction cascades and regulate the cytoskeleton dynamics (Vol. 2 – Chap. 6. Cell Motility). Several signaling pathways are involved in sensing chemoattractant gradient and amplification, among which is the phosphatidylinositol 3-kinase axis.
In addition to migration of leukocytes during inflammation, cell subpopulations continuously patrol organ microvasculature at rest to search for invading particles. Subpopulations ofmonocytes permanently scout the skin, mesentery, and brain microcirculation. Upon infection, they are the very first cells to enter tissue. Intravascular CD1d-restrictedinvariant natural killer T cells patrol within liver sinusoids at a speed of 10 to 20 μm/min [1444]. Vascular patrolling CSF1R + , Ly6g − (in Mus musculus) monocytes are also able to extravasate [1445].
Vascular inflammation is detected by augmented circulating interleukin-6 that is produced by activated inflammatory cells within the vessel wall. Synthesis of IL6, in turn, causes the expression of hepatic acute-phase reactants, such as C-reactive protein, γ-fibrinogen, and angiotensinogen [1446].
11.5.1 Extravasation
Alarm signals generated at inflammatory foci reach the vascular lumen to attract diverseleukocyte subsets that decipher and integrate these signals. Soluble factors, i.e., cytokines, chemokines, chemoattractants, and adhesion molecules as well as adhesion and chemotactic receptors in both endothelial cells and leukocytes, orchestrate various steps of leukocyte recruitment during inflammation. Cytokines and chemokines locally released by an inflammatory focus reach the surrounding endothelium, which becomes activated.
The repertoire of adhesion molecules expressed by different populations of leukocytes as well as chemoattractants and their receptors determines their recruitment. These molecules follow a spatiotemporal organization at the wetted (luminal) surface of the vascular endothelium and leukocyte membrane or create chemical gradients in damaged tissue.
Margination allows circulating leukocytes to move away from the blood stream. Capture, or tethering, represents the first contact of a leukocyte with the activated endothelium. During the first steps — tethering and rolling, labile contacts between leukocytes and activated endothelial cells depend on selectins and their ligands. Once leukocytes are captured, they transiently adhere to the endothelium and begin to roll.28 Rolling allows leukocytes to search for cues such as glycosaminoglycan-immobilized chemokines on the endothelial surface [1445]. Trapped chemokines activate leukocyte chemokine receptors to express leukocyte integrins that bind endothelial ligands for firm adhesion.
During the transition from rolling to firm adhesion and crawling, leukocytes undergo a morphological change from round to polarized promigratory shape.Endothelial cells emit docking microvilli around adhered leukocytes to prevent their detachment. Leukocyte crawl on the endothelium to find a suitable place for transmigration. Crawling includes cell polarization with a leading edge (pseudopod) and a trailing side (uropod), associated with cytoskeletal rearrangement within cell protrusion and retraction. The contractile force that pulls the uropod results from stress fibers with linked myosin-2 nanomotor. Afterward, leukocytes migrate across endothelium if a chemoattractant is present (chemotactic transmigration).
Mechanotaxis, i.e., response to hemodynamical forces, influences crawling. In the absence of flow, neutrophils crawl in all directions. Once shear is applied, neutrophils move perpendicularly to exerted shear [1444].
After transendothelial migration between adjacent endothelial cells (paracellular passage) or across them (transcellular diapedesis), leukocytes traverse the endothelial basement membrane. They then continue to travel through the extracellular matrix guided by a chemotactic gradient.
11.5.2 Molecular Basis of Extravasation
When an inflammatory zone occurs, surrounding endothelial cells become activated by cytokines and chemokines. They increase their content of adhesion molecules on their luminal surface. Extravasation during inflammation occurs preferentially at postcapillary venules.
11.5.2.1 Pro-Inflammatory Cytokines Involved in Extravasation
Leukocyte extravasation is influenced by: (1) pro-inflammatory cytokines, such asinterleukins IL1α, IL1β, and IL6, andTNFα, among others, and (2) chemoattractants for myeloid cells, such as Nformyl methionyl leucyl phenylalanine (fMLP),complement component C5a,leukotriene LTb4, andplatelet-activating factor, produced in the inflammatory site [1445].
The secretion of a selective set of chemokines and upregulated expression of endothelial adhesion molecules caused by specific cytokines direct the subpopulations of leukocytes that are recruited during different phases of inflammation. In response to pro-inflammatory stimuli, such as TNFα, IL1β,Ifnγ, andthrombin,endothelial cells synthesize differentchemokines, such as CCL2, CCL5, CXCL1, CXCL8, CXCL10, or CXCL12 [1445].
Endothelial cells produce chemokines attached to glycosaminoglycans and Duffy receptor for chemokines, especially in apical endothelial microvilli [1445]. These endothelium-immobilized chemokines promote the transition from selectin-mediated tethering and rolling of leukocytes to integrin-mediated firm attachment and spreading, as they activate their cognate Gi/o-coupledchemokine receptors to express leukocyteintegrins. In addition,neutrophils secrete chemoattractants to recruit other leukocyte types in later stages of inflammation.
During inflammation, myeloid cell subpopulations, in which several chemokine receptors are upregulated, migrate from the bone marrow to inflammation loci and uptake antigens. The innate immune response that involvesmonocytes,macrophages, anddendritic cells, occurs in the inflamed tissue. Migration of immature dendritic cells to inflammation zones depends on the expression of chemokine receptors such as CCR2, CCR5, and CCR6 [1445]. After antigen uptake, the density of these chemokine receptors on mature dendritic cells decays, whereas CCR7 expression rises.
Conventional dendritic cells can then move via lymphatics to draining lymph nodes. In lymph nodes, mature dendritic cells present antigens to specific naive T cells. Naive T lymphocytes differentiate into effector(TH1, TH2, TH9, TH17, TH22, and follicular T cells) andregulatory T lymphocytes (Table 11.5). Effector CD4 + T cells, TReg ,cytotoxic CD8 + T cells, andNK cells upregulate chemokine receptors to also migrate to inflammation sites and mount a specialized immune response. In addition, Ly6chigh 29 Ly6g + 30 monocytes migrate to participate in mucosal immunity [1445].
Table 11.5
Chemokine receptors and other products of subpopulations of T lymphocytes (Source: [1445]; Chap. 3; CTL: cytotoxic T lymphocyte; NK: natural killer cell; TFH : follicular helper T lymphocyte; TH: helper T lymphocyte; TReg : regulatory T lymphocyte; Ifn: interferon; IL: interleukin; TNF: tumor-necrosis factor). TH2 cells express chemoattractant receptor homologous molecule expressed in TH2 cells (CRTH2; a.k.a. prostaglandin-D2 receptor [DP2], G-protein-coupled receptor GPR44, and CD294). The synthesis of TH2 cytokines is enhanced by CCL2 and CXCL4 chemokines. TFH cells localize to the follicular regions of B cells owing to chemokine receptor CXCR5 and its ligand CXCL13 secreted by follicular stromal cells. TReg cells modulate the immune response to maintain immune tolerance. They reside mainly in the bone marrow owing to CXCR4 chemokine receptor. During inflammation, they migrate using CCR2, CCR4 to CCR6, and CXCR3 chemokine receptors. CD8 + cytotoxic T cells (CTL) migrate to tissues where CCL3 and CCL4 are synthesized. NK cells upregulate CCR7 to migrate in response to CCL9 and/or CCL21 chemokine.
T lymphocyte | Chemokine | Adhesion | Synthesized |
---|---|---|---|
type | receptors | molecules | cytokines and |
chemokines | |||
TH1 | CCR5/7, | ICAM1, | CCL5 |
CXCR3/4/6 | PSGL1 | IL1/2, 2Ifnγ, | |
CX3CR1 | TNFSF1/2 | ||
TH2 | CCR4/7/8 | IL4/5/10/13 | |
CXCR4 | |||
TH9 | IL9/10 | ||
TH17 | CCR2/4 (at rest), | CCL20 | |
CXCR4/6 (at rest) | IL17/21/22/26 | ||
CCR6 (activated) | |||
TH22 | CCR4/6/10 | IL22 | |
TFH | CXCR5 | IL21 | |
TReg | CXCR4 (at rest), | IL10, | |
CCR2/4/5/6/7, | TNFSF4/5 | ||
CXCR3 (migration) | |||
CD8 + CTL | CCR5 | ||
NK | CCR7 |
11.5.2.2 Adhesion Molecules Involved in Extravasation
Numerousadhesion molecules are implicated in transmigration, such as: (1) selectins (E-, L-, and P-selectins);31 (2) cadherins such as vascular endothelial (VE)-cadherin, or cadherin-5; (3) immunoglobulin-like adhesion molecules, such as platelet–endothelial cell adhesion molecule PECAM1, intercellular adhesion molecules (e.g., ICAM1 and ICAM2), and vascular cell adhesion molecule VCAM1; (4) integrins, such as α4β1-, α4β7-, αLβ2-, and αMβ2-integrin; as well as (5) integrin-associated protein, or leukocyte surface antigen CD47 (Table 11.6; Vols. 1 – Chap. 7. Plasma Membrane and 2 – Chap. 6. Cell Motility). Leukocyte integrins connect to their endothelial ligands that are organized in tetraspanin-enriched microdomains [1445]. These cellular structures mediate the contact between leukocytes and endothelial cells during extravasation.
Table 11.6
Adhesion molecules involved in leukocyte migration across blood vessel wall during inflammation (Source: [1447, 1448]; GAGCAM: glycosaminoglycan cell adhesion molecule, or glycosylation-dependent CAM [GlyCAM1]); ICAM: intercellular adhesion molecule; MAdCAM: mucosal vascular addressin cell adhesion molecule; PECAM: platelet endothelial cell adhesion molecule; VCAM: vascular cell adhesion molecule). 3-fucosyl Nacetyl lactosamine (FAL; a.k.a. Lewis X or CD15) is a glucid adhesion molecule expressed on glycoproteins and -lipids as well as proteoglycans that is synthezised by fucosyltransferases FuT4 and FuT9. It mediates neutrophil chemotaxis and phagocytosis. The random contact is followed by rolling to a stop involving selectins. Sticking and extravasation require integrins.
Extravasation step | Adhesion molecules |
---|---|
Rolling | Selectins (CD62E/L/P), FAL (CD15), PSGL1 (CD162) |
GAGCAM1, neutrophil addressins | |
Stopping | α4β1-, β2-, α4β7-, αLβ2-integrins |
ICAM1, VCAM1, MadCAM1 | |
Transmigration | β2-integrins |
ICAM1, VCAM1, PECAM1 |
Endothelial adhesion receptors of activated endothelial cells localize to adhesion platforms that are enriched in tetraspanins in apical microvilli. Tetraspanins interact simultaneously with other tetraspanins and other types of transmembrane receptors. These adhesion platforms contain selectins and members of the Ig superfamily (VCAM1, ICAM1, ICAM2, PECAM1, and JAMs) [1445]. These functional units that coalesce around adherent leukocytes are connected to the actin cytoskeleton and contain α-actinin, ezrin, moesin, paxillin, talin, vinculin, and vasoactive stimulatory phosphoprotein. They are regulated by the Rho–RoCK pathway and PIP2 second messengers. These docking structures, or transmigratory cups, stabilize firm adhesion of leukocyte to endothelium, hence preventing leukocyte detachment.
During leukocyte displacement over the endothelium, L-selectin, epican (CD44), ICAM1, and ICAM3, among others redistribute to the leukocyte rear pole. On the oher hand, integrins, such as αLβ2– and αMβ2-integrins, and chemokine receptors localize to the leading edge and endothelial contact area.32
Leukocytes probe for sites suitable for diapedesis by constructing protrusions. When they find an appropriate site for migration, filopodia evolve into an invasive pseudopod to cross the endothelium. During paracellular transmigration, ICAM1 and JAMa interact with αLβ2-integrin and form a ring-shaped cluster around the leukocyte [1445]. Transcellular migration requires the supply of additional membrane by the vacuolovesicular organelles to build a transcellular pore that lenghtens toward the basal membrane. Both ICAM1 and caveolin-1 translocate toward the transcellular pore. Membrane fusion is regulated by Ca2 + and SNARE-containing complexes [1445]. Intermediate filament constituent vimentin contributes to the transcellular pore.
11.5.2.3 Selectins
The selectin family of transmembrane adhesion receptors mediates leukocyte tethering and rolling. Selectin-P on endothelial cells is the primary adhesion molecule for cell capture and rolling onto vascular endothelium. The main leukocyte ligand for P-selectin is selectin-P ligand (SelPLg; a.k.a. P-selectin glycoprotein ligand-1 [PSGL1] and CD162).33
Selectin-L also contributes significantly to leukocyte capture. Selectin-L and -E also take part in rolling. Selectin-L is much less efficient than P-selectin in cell rolling. Nonetheless, it is necessary to capture leukocytes and initiate their rolling. Selectin-E may be responsible for slow rolling interactions and possibly the initiation of firm adhesion.
Selectin-E and -P interact with L-selectin and PSGL1 expressed by leukocytes (Table 11.7). In addition, E-selectin targets other specific ligands, such as E-selectin ligand ESL1 (a.k.a. Golgi body sialoglycoprotein GlG1 and cysteine-rich fibroblast growth factor receptor CFR1), epican (a.k.a. extracellular matrix receptor ECMR3, GP90 lymphocyte homing–adhesion receptor, heparan sulfate proteoglycan receptor, and CD44).
Table 11.7
Adhesion molecules of leukocyte binding to endothelium and their interactions and functions (Source: [1448]; HCAM: homing cell adhesion molecule; I: integrin; TEM: transendothelial migration). High endothelial venules (HEV) are specialized postcapillary venous swellings with cuboidal endothelial cells in all secondary lymphoid organs, except the spleen, that enable circulating leukocytes to enter lymph nodes in any organ, tonsils and adenoids in the pharynx, Peyer’s patches in the small intestine, and small aggregates of lymphoid tissue in the stomach and large intestine, once they have crossed high endothelial venules. Sialyl Lewis antigen X (sLe x ), a carbohydrate E-selectin ligand, is constitutively expressed on granulocytes and monocytes; it mediates their extravasation. It is also expressed by activated T (e.g., TH1, but not TH2 cells) and B lymphocytes.
Neutrophil | Endothelial cell | Role | |
---|---|---|---|
Receptor | Receptor | ||
Selectin | L-selectin, | E/P-selectins | Rolling, |
interactions | sLe x | GAGCAM, | binding to HEV |
neutrophil | |||
addressins | |||
Integrin–IgCAM | αLβ2-I | ICAM1/2, | Adhesion; |
interactions | α4β1-I | VCAM1 | homing |
IgCAM | PECAM1 | PECAM1 | Adhesion potentiation; |
interactions | HCAM | TEM; | |
binding to connective | |||
tissue components |
11.5.2.4 Integrins
β2 Integrins participate in leukocyte deceleration and arrest. Neutrophils express small amounts of other integrins such as α4β1-integrin that binds to endothelial VCAM1 molecule. Integrin-αLβ2 is the most important integrin in firm leukocyte adhesion, whereas αMβ2-integrin seems to be important in neutrophil activation and phagocytosis. Both αLβ2– and αMβ2-integrins can bind to ICAM1 and ICAM2 molecules.
Neutrophils and some lymphocyte subpopulations use preferentially αLβ2-integrin for firm adhesion. On the other hand, monocytes and lymphoblasts target predominantly α4β1-integrin [1445].
11.5.2.5 Immunoglobulin-like Adhesion Molecules
Several integrin ligands, such as vascular cell (VCAM1) and intercellular (ICAM1–ICAM2) adhesion molecules, are involved in firm adhesion, crawling, and paracellular and transcellular migration. Other types of Ig-like adhesion molecules, such as platelet–endothelial cell (PECAM1), junctional (JAM), and endothelial cell-selective adhesion molecule (ESAM), T-cell surface glycoprotein-E2 (or CD99), among others, act during paracellular transmigration [1445] (Table 11.8).
Table 11.8
Endothelial cell junctional molecules in leukocyte transmigration (Source: [1449]). Substance CD99 expressed on all leukocytes is also called T-cell surface glycoprotein-E2. Molecule CD99-like-2 protein (CD99L2) is synthesized in leukocytes and endothelial cells. It blocks neutrophil transmigration through the perivascular basement membrane,
Type | Leukocyte type | Stimulus | Extravasation |
---|---|---|---|
specificity | specificity | stage
![]() Stay updated, free articles. Join our Telegram channel![]() Full access? Get Clinical Tree![]() ![]() ![]() |