Summary
The discovery of angiotensin-I-converting enzyme 2 (ACE2) and a (pro)renin receptor has renewed interest in the physiology of the renin-angiotensin system (RAS). Through the ACE2/angiotensin-(1–7)/Mas counter-regulatory axis, ACE2 balances the vasoconstrictive, proliferative, fibrotic and proinflammatory effects of the ACE/angiotensin II/AT1 axis. The (pro)renin receptor system shows an angiotensin-dependent function related to increased generation of angiotensin I, and an angiotensin-independent aspect related to intracellular signalling. Activation of ACE2 and inhibition of ACE and renin have been at the core of the RAS regulation. The aim of this review is to discuss the biochemistry and biological functions of ACE, ACE2 and renin within and beyond the RAS, and thus provide a perspective for future bioactives from natural plant and/or food resources related to the three proteases.
Résumé
La découverte de l’enzyme de conversion de l’angiotensine 2 (AC2) et un récepteur à la prorénine est une avancée récente dans la compréhension de la physiologie du système rénine-angiotensine. Au sein de l’axe inhibiteur de l’enzyme de conversion de l’angiotensine 2/angiotensine 1/MAS, l’AC2 contrebalance l’effet vasoconstricteur, prolifératif, fibrosant et pro-inflammatoire de l’axe ACE/angiotensine 2/AT1. Le récepteur à la prorénine a une fonction angiotensine dépendante, liée à l’augmentation de la production d’angiotensine 1, et un aspect indépendant de l’angiotensine, lié aux signaux intracellulaires. L’activation de l’AC2 et l’inhibition de l’ACE de la rénine ont été considérées comme au centre de la régulation du système rénine-angiotensine. L’objet de cette revue générale est de discuter les fonctions biochimiques et biologiques de l’ACE, de l’AC2 et de la rénine au sein et au-delà du système rénine-angiotensine et ainsi de proposer une perspective de développement d’agents actifs extraits de plantes naturelles ou d’alimentation, produits liés à ces trois protéases.
Background
The renin-angiotensin system (RAS) is not only an endocrine but also a paracrine and an intracrine system . In mammals, the intravascular RAS plays a key role in maintaining blood pressure homeostasis and fluid and salt balance, and the tissue or local RAS is involved in physiological and pathological processes, such as tissue growth and remodelling, development and inflammation . In a classical RAS, the substrate angiotensinogen (AGT), which is released into the circulation from the liver, is degraded by the enzyme renin that originates in the kidney, generating the inactive angiotensin I (Ang I). When this decapeptide comes into contact with angiotensin-I-converting enzyme (ACE) at the endothelial surface of blood vessels, the C-terminal dipeptide is cleaved, giving rise to angiotensin II (Ang II), the main effector molecule of the RAS. Through interactions with specific receptors, particularly its type 1 or AT1 receptor, Ang II stimulates a wide variety of signalling pathways in the heart, blood vessels, kidneys, adipose tissue, pancreas and brain, initiating most of the physiological and pathophysiological effects that have been attributed to the RAS . Due to the function of directly generating the main effector Ang II, ACE – together with the classical axis ACE/Ang II/AT1 – has been at the core of RAS studies since its discovery.
In 2000, a homologue of ACE, known as angiotensin-I-converting enzyme 2 (ACE2), was cloned by two independent research groups . Evidence indicates that ACE2 negatively regulates the activated RAS by degrading Ang II to the heptapeptide Ang-(1–7). Moreover, through the Mas receptor (a G protein-coupled receptor), the resulting Ang-(1–7) counterbalances the cardiovascular effects of Ang II by opposing many AT1 receptor-mediated actions . ACE2 and the axis ACE2/Ang-(1–7)/Mas are becoming the focus of intense research regarding the RAS . The discovery of a (pro)renin receptor ([P]RR) and the introduction of renin inhibitors have also brought (pro)renin back into the spotlight . Far from being a straightforward cascade containing one substrate (AGT), two proteases (renin and ACE), two peptides (Ang I and Ang II) and one receptor (AT1), the RAS currently consists of several axes upstream and downstream of the classical cascade, which include more than two dozen peptidases, nearly a dozen Ang fragments and at least six different receptors . Here, we review three critical proteases (ACE, ACE2 and renin) within and beyond the RAS and thus intend to find new connections between natural plant and/or food resources and the RAS.
Occurrence, gene encoding and structure of ACE
ACE (EC 3.4.15.1) is a monomeric glycoprotein that is distributed in many tissues and biological fluids. There are two isoforms of ACE in humans: somatic ACE (sACE) and germinal ACE (gACE). Somatic ACE is found in many types of endothelial and epithelial cells . Germinal ACE or testicular ACE is present exclusively in germinal cells in the male testis. Although ACE is a type I integral membrane protein, it can also be released as a soluble enzyme into extracellular fluids, such as plasma and seminal and cerebrospinal fluids, following post-translational proteolytic cleavage by a membrane protein sheddase or secretase .
Somatic ACE and gACE are encoded by a single gene containing 26 exons. The promoter for sACE is situated in the 5′ flanking region of the first exon, whereas that for gACE is within intron 12, which results in different lengths for the two isoforms. The longer sACE (150–180 kDa) is transcribed from exon 1 to exon 26, excluding exon 13, whereas the shorter gACE (90–110 kDa) is transcribed from exon 13 to exon 26. Exon 13 encodes a unique sequence for the N-terminus of gACE, whereas downstream exons encode a common sequence for both isozymes .
Somatic ACE and gACE both consist of a 28-residue hydrophilic C-terminal cytoplasmic domain, a 22-residue hydrophobic transmembrane domain that anchors the protein in the membrane and an N-terminal ectodomain ( Fig. 1 ) that is heavily glycosylated with mannose, galactose, fructose, N-acetylneuraminic acid and N-acetylglucosamine . The ectodomain of sACE is further divided into two similar domains (N domain and C domain) encoded by the homologous exons 4–11 and 17–24, respectively, and each domain contains an active His-Glu-X-X-His (HEXXH) sequence . Somatic ACE is the only known metallopeptidase with two homologous active sites , which implies that there has been a gene duplication event during evolution . Except for a unique sequence constituting its N-terminus, gACE is identical to the C-terminal half of sACE . Due to cleavage of the membrane-bound residues by ACE secretase, soluble circulating ACE lacks a transmembrane portion and a cytosolic domain .

The three-dimensional X-ray crystallographic structure of a deglycosylated truncated version of gACE (C domain of sACE), reveals a preponderance of α-helices with a zinc ion and two chloride ions incorporated. A deep narrow channel separates the molecule into two subdomains and the active site is located toward the bottom of this channel. An N-terminal ‘lid’ on the top of molecule appears to allow only small peptide substrates access to the active site cleft. In fact, the structure bears little similarity to that of carboxypeptidase A (M14 family) on which the initial drug development of ACE inhibitors was based. Instead, it resembles rat neurolysin (M3 family) and Pyrococcus furiosus carboxypeptidase (M32 family), despite sharing little sequence similarity with these two proteins . Corradi et al. reported the crystal structure of the N domain of sACE. Similarly, it has an ellipsoid shape with a central groove dividing it into two subdomains, one of which contains the N-terminal region that covers the central binding cavity. But the structure reveals differences in the active site and it contains only one chloride ion, equivalent to chloride II of gACE. The three-dimensional structures of C domains (based on gACE) and N domains provide an opportunity to design domain-selective ACE inhibitors that may exhibit new pharmacological profiles .
Catalytic mechanism of ACE
According to the catalytic mechanism and the critical amino acid residue involved, peptidases are classified into four major types: serine, cysteine, aspartic and metallo . ACE is an M2 family metallopeptidase: MA(E), the gluzincins . Two histidine residues of the functional motif HEXXH and a third distant glutamate positioned 23–24 residues further towards the C-terminus are the ligands for the zinc cofactor . An activated water molecule complexed to Zn 2+ serves as the nucleophile to attack the carbonyl group of the targeted peptide bond .
The activity of ACE is also chloride dependent. Chloride primarily activates the active sites of ACE and enhances the binding of substrates . Each active domain of ACE displays differences in sensitivity to chloride activation . The activity of the C domain of sACE depends highly on chloride ion concentration and is inactive in its absence, whereas the N domain can be completely activated at relatively low concentrations of this anion and is still active in the absence of chloride . Germinal ACE depends on chloride to a lesser extent compared with the C domain of sACE . Cushman and Cheung reported an optimal in vitro ACE activity of rabbit rung acetone extract in the presence of 300 mM NaCl at pH 8.1–8.3.
The two active domains of sACE are also subtly different in substrate specificity. They hydrolyze bradykinin almost equally but the C domain active site can hydrolyze Ang I, substrate P and Hippuryl-His-Leu more efficiently, while the N domain active site preferentially hydrolyzes Ang-(1–7) , luteinizing hormone-releasing hormone (LH-RH) , the haemoregulatory peptide N-acetyl-Ser-Asp-Lys-Pro (AcSDKP) and Alzheimer amyloid β-peptide (Aβ) . Fuchs et al. proved that the C-terminal catalytic domain was the main site of Ang I cleavage in mice. The differentiation of catalytic specificity might be due to very subtle variation in substrate-specific amino acids and chloride-induced conformational alteration of active sites .
ACE acts as an exopeptidase to cleave dipeptides from the free C-termini of two typical substrates, Ang I and bradykinin. For certain substrates such as cholecystokinin , substrate P and LH-RH , which have amidated C-termini, ACE not only displays exopeptidase activity but also acts as an endopeptidase . The most prominent example of endopeptidase activity is ACE hydrolyzing the synthetic Aβ-(1–40) peptide into four fragments: an Aβ-(8–40) peptide and the others corresponding to products of Aβ-(1–7) hydrolysis . Thus, ACE might have a more general impact on the metabolism of biologically active peptides than previously recognized . The two substrates used most often for measuring ACE activity and inhibition in vitro – Hippuryl-His-Leu and N-[3-(2-furyl)acryloyl]-L-phenylalanylglycylglycine (FAPGG) – only have the N-termini blocked and substrates with two termini blocked have been developed .
Biological impact of ACE
ACE was originally isolated in 1956 as a ‘hypertensin-converting enzyme’ . In the RAS, ACE cleaves the decapeptide Ang I-(1–10) (Asp-Arg-Val-Tyr-Ile-His-Pro-Phe-His-Leu) into the octapeptide Ang II-(1–8) by removing the C-terminal dipeptide His-Leu. When the RAS is overactive, Ang II exerts its harmful effects primarily via the AT1 receptor, whereas the AT2 receptor may oppose and counterbalance those effects mediated by AT1 receptor to exert protective actions . Ang II is a potent vasoconstrictor, stimulates the release of aldosterone and antidiuretic hormone or vasopressin and increases the retention of sodium and water. These effects act directly in concert to raise blood pressure. A nonapeptide derivative of Ang I, des-Asp1-Ang I-(2–10), prevents infarction-related and non-infarction-related cardiac injuries and disorders. His-Leu can be cleaved from the peptide by ACE to produce Ang III-(2–8) , which has 40% of the vasoconstriction activity of Ang II. Ang III exerts its effects, in principle, in a similar manner to Ang II, and may be equally or even more important in mediating the release of vasopressin . ACE also degrades Ang-(1–9) to Ang-(1–7) then further degrades this peptide to the inactive Ang-(1–5) ( Fig. 2 ). In addition, ACE (also termed kininase II) inactivates the vasodilators bradykinin-(1–9) (Arg-Pro-Pro-Gly-Phe-Ser-Pro-Phe-Arg) and kallidin (Lys-bradykinin) in the kallikrein-kinin system, by cleaving the C-terminal dipeptide Phe-Arg. ACE eventually cleaves its primary metabolite bradykinin-(1–7) into the shorter fragment bradykinin-(1–5) .

Through Ang II and aldosterone, ACE may also be implicated in the impairment of nitric oxide bioavailability and cell oxidative stress, augmenting the generation of reactive oxygen species and peroxynitrite . With the ability to hydrolyze neuropeptides such as enkephalin , substrate P, neurotensin and LH-RH, ACE may be involved in the functioning of the brain and nervous system. ACE may affect the digestive system by hydrolyzing the peptide hormone cholecystokinin and gastrin . The in vivo experiment conducted by Azizi et al. proved that acute ACE inhibition could increase the level of the natural stem cell regulator AcSDKP in plasma. AcSDKP substantially inhibits cell cycle entry of normal haematopoietic stem cells and protects haemopoiesis against damage caused by cycle-active cytotoxic agents . AcSDKP can also inhibit the proliferation of hepatocytes and lymphocytes and stimulate angiogenesis in vivo. The in vivo antifibrotic effect of ACE inhibition is partially mediated by AcSDKP . ACE may also affect susceptibility to Alzheimer’s disease by degrading Aβ and preventing the accumulation of amyloid plaques in vivo . In the brains of amyloid precursor protein Swedish mutation transgenic mice, ACE converts Aβ 1–42 to Aβ 1–40 and degrades Aβ, and chronic inhibition of ACE with captopril enhances predominant Aβ 1–42 deposition . However, through the inhibition of brain ACE activity in the Aβ 25–35 -injected mice, perindopril ameliorates cognitive impairment and may therefore have a beneficial effect on Alzheimer’s disease as well as hypertension .
ACE inhibitors
Using the assumed mechanistic analogy to other zinc metallopeptidases, plus the knowledge that several snake-venom peptides potentiate the action of bradykinin by inhibiting ACE, efforts were undertaken to develop orally-active peptide analogues for potential use in the treatment of hypertension . The first such compound, captopril or D-3-mercapto-2-methylpropanoyl-L-proline, is an analogue of the Ala-Pro sequence, with sulphydryl as a strong chelating group for the zinc ion. Its adverse effects, which were the same as those caused by mercapto-containing penicillamine, prompted the design of non-sulphydryl ACE inhibitors . The results were two active inhibitors: enalaprilat and lisinopril. They are both essentially tripeptide analogues with a zinc-co-ordinating carboxyl group and a phenylalanine that occupies the S1 groove in the enzyme. Lisinopril is a lysine analogue of enalaprilat but it is hydrophilic, with greater affinity than enalaprilat. The later compounds are all variations of the first three inhibitors, with most of the differences residing in the functionalities that bind the active site zinc and the S2′ pocket. In addition to phosphonates, ketones are also useful as chelators .
Currently, there are more than 10 ACE inhibitors marketed that are widely used as first-line therapy for cardiovascular diseases, including hypertension, heart failure, heart attack and left ventricular dysfunction. According to the functional moiety, they are divided into three types: thiol (captopril), carboxylate (benazepril, enalapril, lisinopril, moexipril, perindopril, quinapril, ramipril, trandolapril) or phosphate (fosinopril). Some ACE inhibitors are now administrated clinically as ethyl-ester prodrugs, which have good bioavailability but are inactive in their own right. They are then converted to the active diacid molecules in vivo by esterases.
As a drug class, ACE inhibitors are very effective, have a relatively low incidence of side effects and are well tolerated. A common side effect of ACE inhibitors is a dry cough, which appears in 5–20% of patients and may result in the discontinuation of treatment. Another serious problem is angioedema, which affects 0.1–0.5% of patients and can be life-threatening. The two side effects have generally been attributed to altered concentrations of bradykinin . Use of ACE inhibitors during the second and third trimesters of pregnancy is contraindicated because of their association with an increased risk of foetopathy, a group of conditions that includes oligohydramnios, intrauterine growth retardation, hypocalvaria, renal dysplasia, anuria, renal failure and death. Exposure to ACE inhibitors during the first trimester of pregnancy may place the infant at increased risk for major congenital malformations .
The initial drug development of clinical ACE inhibitors was based on the assumption of an active site related to that of carboxypeptidase A but organized to remove a dipeptide rather than a single amino acid from the C-terminus of its substrate. It is now known that sACE has two active sites, neither of which resembles that of carboxypeptidase A, and that these sites are not identical. Clinical ACE inhibitors, however, show little discrimination between these two active sites . Ang I is hydrolyzed predominantly by the C domain of sACE in vivo but bradykinin is hydrolyzed by both active sites ; therefore a C domain-selective inhibitor would allow some degradation of bradykinin by the N domain and this degradation could be enough to prevent the accumulation of excess bradykinin that has been observed during attacks of angioedema. That is, the C domain-selective inhibition could possibly result in specialized control of blood pressure with fewer vasodilator-related adverse effects . A structure-activity study has proved that the group substitution involving the phenyl ring and carbon chain at the sulphonyl and propionyl moieties of captopril is essential for better activity towards the C domain of ACE . There is increasing evidence that the N domain of sACE is responsible for the in vivo degradation of the natural haemoregulatory hormone AcSDKP . So, N domain-selective inhibition might open up novel therapeutic areas. Two phosphinic tetrapeptides, RXPA380 and RXP407, have been found to be highly selective inhibitors of the C and N domains of sACE, respectively . The availability of the three-dimensional structures of the C and N domains of sACE makes the structure-based design of active site-specific inhibitors possible .
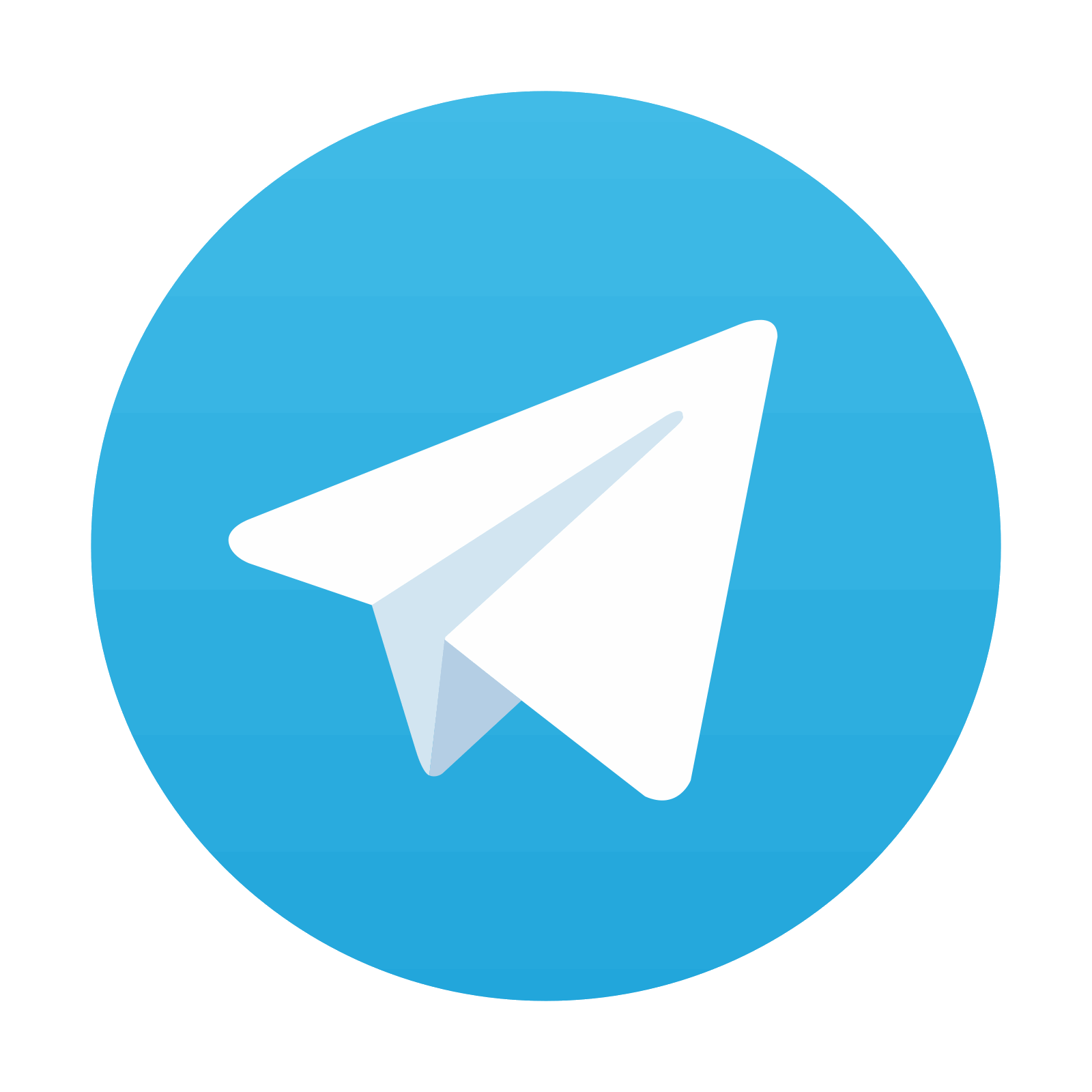
Stay updated, free articles. Join our Telegram channel
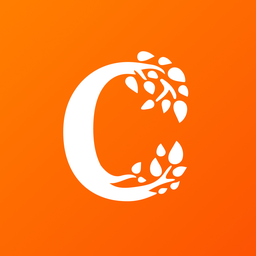
Full access? Get Clinical Tree
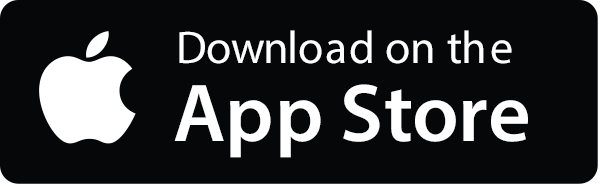
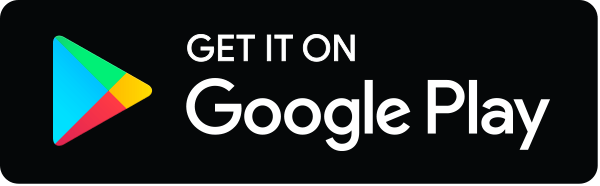