Background
With the advent of three-dimensional (3D) printers and high-resolution cardiac imaging, rapid prototype constructions of congenital cardiac defects are now possible. Typically, source images for these models derive from higher resolution, cross-sectional cardiac imaging, such as cardiac magnetic resonance imaging or computed tomography. These imaging methods may involve intravenous contrast, sedation, and ionizing radiation. New echocardiographic transducers and advanced software and hardware have optimized 3D echocardiographic images for this purpose. Thus, the objectives of this study were to confirm the feasibility of creating cardiac models from 3D echocardiographic data and to assess accuracy by comparing 3D model measurements with conventional two-dimensional (2D) echocardiographic measurements of cardiac defects.
Methods
Nine patients undergoing 3D echocardiography were identified (eight with ventricular septal defects, one with three periprosthetic aortic valve leaks). Raw echocardiographic image data were exported anonymously and converted to Digital Imaging and Communications in Medicine format. The image data were filtered for noise reduction, imported into segmentation software to create a 3D digital model, and printed. Measurements of the defects from the 3D model were compared with defect measurements from conventional 2D echocardiographic data. Meticulous care was taken to ensure identical measurement planes.
Results
Long- and short-axis measurements of eight ventricular septal defects and three perivalvar leaks were obtained. Mean ± SD values for the 3D model measurements and conventional 2D echocardiographic measurements were 7.5 ± 6.3 and 7.1 ± 6.2 mm respectively ( P = .20), indicating no significant differences between the standard 2D and 3D model measurements. The two groups were highly correlated, with a Pearson correlation coefficient of 0.988. The mean absolute error (2D − 3D) for each measurement was 0.4 ± 0.9 mm, indicating accuracy of the 3D model of <1 mm.
Conclusions
Three-dimensional printed models of echocardiographic data are technically feasible and may accurately reflect ventricular septal defect anatomy. Three-dimensional models derived from 3D echocardiographic data sets represent a new tool in procedural planning for children with congenital heart disease.
Highlights
- •
Three-dimensional models can display congenital heart defects created from 3D cardiac imaging.
- •
Three-dimensional echocardiographic images can be used to create 3D models of the heart.
- •
Three-dimensional echocardiography–derived digital models demonstrate defects accurately when compared with standard 2D echocardiographic imaging.
The diagnosis and management of structural and congenital heart disease is driven largely by two-dimensional (2D) echocardiography because of its portability, safety, high spatial and temporal resolution, particularly in infants and children, and ease of use. However, congenital heart disease is a three-dimensional (3D) problem, so 2D imaging methods often lack critical spatial information. New echocardiographic transducers and advanced software and hardware have significantly improved 3D echocardiographic images. Three-dimensional echocardiographic information preserves 3D spatial relationships but still presents challenges in the display of the 3D image information.
Three-dimensional printing technology has significantly advanced in the past 10 years. Three-dimensional printers now have the capability to print at any scale from nearly any type of material. They have been used in various biomedical applications from prosthesis manufacture to cell and tissue culture. They have incredible spatial resolution to pick up on microscopic details. There is an emerging field involving the use of 3D simulation data in education and preprocedural planning, demonstrating excellent results in general, including the cardiology and cardiovascular surgery fields. Typically, cardiac magnetic resonance imaging or computed tomographic data are used to print high-quality cardiac reconstructions, which may involve intravenous contrast, sedation, and ionizing radiation. There are no reports of 3D printing of echocardiography-based data sets. Ultrasound provides several advantages over cardiac magnetic resonance imaging and computed tomography: it is portable, is readily available, possesses high temporal resolution, involves no radiation, and can be done without sedation or with conscious sedation when indicated.
Thus, our aim was to utilize the newest advances in 3D echocardiography in conjunction with state-of-the-art image segmentation software to create printed 3D models of structural congenital heart disease and compare measures derived from those models with corresponding measures from conventional 2D echocardiographic images.
Methods
In an institutional review board–approved study, nine patients with structural heart disease undergoing 3D echocardiography before intracardiac repair were identified (eight with ventricular septal defects [VSDs], one with three perivalvar leaks around a prosthetic aortic valve). Images were acquired with the Philips X7-2 (Philips Medical Systems, Andover, MA) for transthoracic imaging from the subcostal view, with fused frequencies ranging from 2 to 7 MHz. For the patient with perivalvar leaks, images were acquired with the Philips X7-2t probe in the home view at the level of the aortic valve. Three-dimensional echocardiographic acquisition parameters were optimized during imaging to produce a full data set with optimized spatial resolution. Elevation width was maximized to ensure full coverage of the cardiac defects, and image depth was minimized to optimize frame rate and temporal resolution. Additionally, the loop acquisition was dialed down to acquire in diastole only, which typically yielded four or five frames of data, depending on the frame rate and heart rate. Image gains were set at the minimal level to achieve desired topography of the defect while minimizing noise in the blood pool and at the periphery of the 3D data set.
Once acquired, all frames were reviewed for quality and clarity in the 3D image analysis software (QLAB Cardiac Analysis version 9; Philips Medical Systems), and the optimal 3D echocardiographic image time point was selected. Raw echocardiographic image data were exported anonymously, and the single 3D image time point data were converted to Digital Imaging and Communications in Medicine (DICOM) format. The image data were filtered using filters for noise reduction. Specifically, the nonlocal means image filter was used to despeckle the blood pool while maintaining important anatomic details. Figure 1 demonstrates a short-axis slice from a 3D echocardiographic data set before and after filtering. Using specialized segmentation software (Mimics; Materialise, Leuven, Belgium), a 3D digital replica of the heart, centered on the structure of interest, was created. Various segmentation methods, including automatic, semiautomatic, and hand segmentation methods, were used to create an accurate 3D digital cardiac segmentation. The completed cardiac segmentation was exported as an STL file and prepared for printing at a 1:1 scale using an Objet500 Connex Polyjet printer (Stratasys, Eden Prairie, MN). Figure 2 demonstrates the entire model creation process whereby a digital model was created from segmentation of a 3D echocardiographic dataset and then printed.


Measurements of the defects (VSDs or perivalvar leaks) in two nearly orthogonal planes were taken from the 3D digital model using postprocessing software to select accurate planes (3Matic; Materialise). Three-dimensional measurements were performed on the 3D digital model instead of the 3D printed model, because it was easier to replicate the plane of the 2D echocardiographic measurement, it was easier to get a true defect measurement around the curves of the printed model, and the 3D digital and printed models are accurate to 30 μm ( Figure 3 ). These measurements were compared with defect measurements from conventional 2D echocardiographic data ( Figures 4 and 5 ). Meticulous care was taken to ensure identical measurement planes on the 2D echocardiographic and 3D digital segmentation data sets. Peripheral features of the image that give a sense of how anterior and posterior the long-axis measurement was located (i.e., presence of the aortic valve in the frame, presence of the septal leaflet of the tricuspid valve in the frame, presence of the coronary sinus in the frame, etc) and how superior and inferior the short-axis measurement was located (i.e., the presence of the moderator band, aortic valve leaflets, etc) were used to ensure that the 3D digital measurement and the 2D echocardiographic measurement were in the exact same plane. Additionally, all measurements were taken on the 2D images in the same portion of the cardiac cycle, typically end-diastole, as the 3D digital segmentation.



Measurements were tabulated and compared with means and standard deviations. Absolute error for each measurement was also calculated as the absolute value of the 2D − 3D measurement, and mean error and standard deviation were calculated. A Bland-Altman analysis was performed for agreement between 2D and 3D measurements.
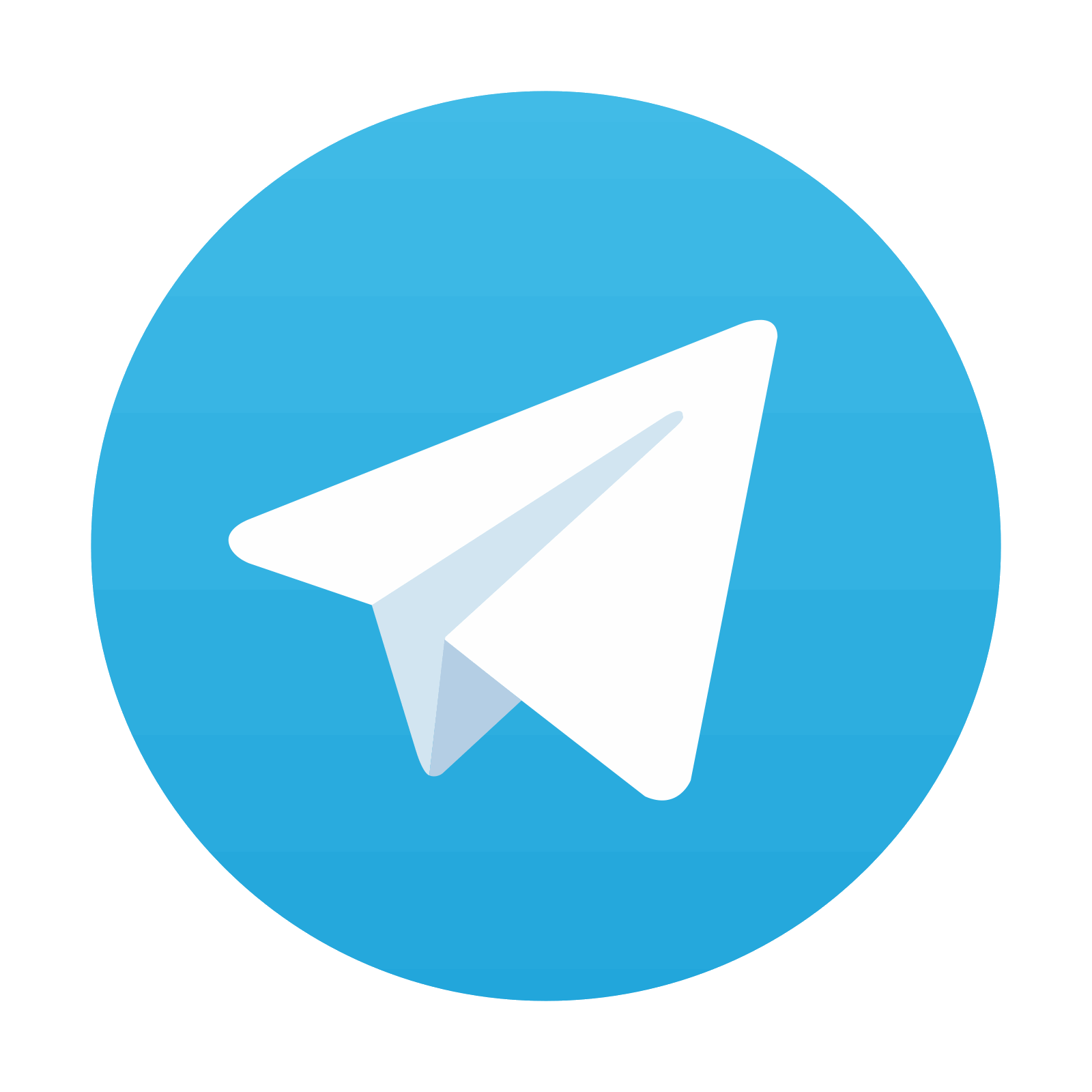
Stay updated, free articles. Join our Telegram channel
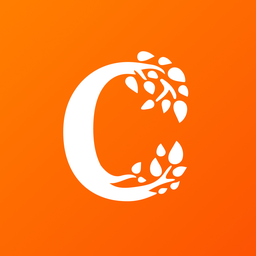
Full access? Get Clinical Tree
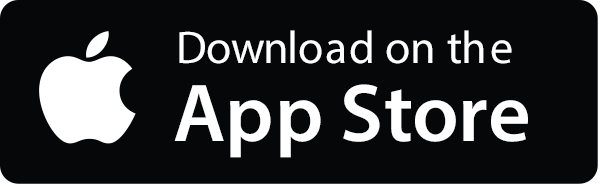
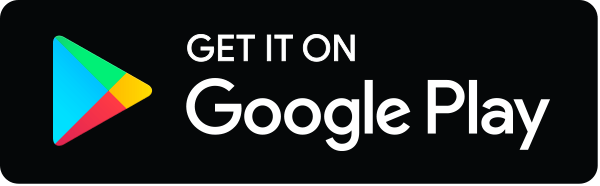