Background
Right ventricular (RV) volume and functional assessments are essential in the management of pulmonary arterial hypertension but are often difficult to perform. Three-dimensional (3D) echocardiography is limited by acoustic dropout of the RV free wall in dilated ventricles. The aim of this study was to test the hypothesis that knowledge-based reconstruction, a novel method for 3D modeling of RV endocardium from two-dimensional echocardiographic images, could provide accurate measurements of RV volumes and systolic function.
Methods
Twenty-seven patients with pulmonary arterial hypertension were prospectively recruited for same-day echocardiography and cardiovascular magnetic resonance (CMR), which was used as a reference standard. Two-dimensional transthoracic echocardiographic images were acquired with 3D spatial localization equipment to allow 3D reconstruction. Image analysis was performed with dedicated software to obtain end-diastolic volume (EDV) and end-systolic volume (ESV) and RV ejection fraction (EF). The method of disks was used to determine RV volumes on CMR.
Results
Echocardiographic RV volumes correlated well with CMR (EDV, R = 0.87; ESV, R = 0.88; EF, R = 0.75). For interobserver analyses, coefficients of variability were 7.8 ± 7.0% for EDV, 10.2 ± 8.0% for ESV, and 15.4 ± 13.8% for EF. For intraobserver analyses, coefficients of variability were 7.1 ± 5.1% for EDV, 8.3 ± 7.0% for ESV, and 10.9 ± 9.2% for EF. On Bland-Altman analyses, volumes obtained on transthoracic echocardiography (TTE) were slightly larger than those obtained by CMR (ΔEDV TTE-CMR , 5.8 ± 33.7 mL; ΔESV TTE-CMR , 3.5 ± 27.8 mL), whereas EFs tended to be slightly higher by CMR (ΔEF CMR-TTE , 0.5 ± 6.5%).
Conclusions
Knowledge-based reconstruction provides accurate and reproducible measurements of RV volumes in patients with pulmonary arterial hypertension. Larger studies are needed to confirm these results and to determine the practicality of this approach in daily practice and as an end point in clinical trials.
Transthoracic echocardiography (TTE) is the most commonly used modality for the assessment of right ventricular (RV) size and systolic function. As a result of the complex shape of the right ventricle, simple geometric modeling to obtain RV volumes and ejection fractions (EFs) by two-dimensional (2D) TTE is difficult. Three-dimensional (3D) TTE correlates considerably better with the reference standard of cardiovascular magnetic resonance (CMR), but image quality is often suboptimal. Particularly in patients with dilated right ventricles, the exclusion of the free wall from the imaging sector frequently leads to inaccuracy of RV volumes. Given the limitations of standard 2D TTE and 3D TTE, alternative techniques for noninvasive assessment of RV size and function are needed in clinical practice. This is particularly true for the evaluation and management of patients with dilated ventricles, such as patients with pulmonary hypertension.
The generation of a 3D RV model from 2D transthoracic echocardiographic images is possible with knowledge-based reconstruction (KBR), a method that has previously been validated in vitro and against CMR in patients with congenital heart disease. This technique involves the acquisition of 2D images localized in 3D space by a magnetic field generator, located under the patient’s bed, and a magnetic field sensor, attached to the echocardiographic transducer. The user identifies specific anatomic landmarks, and the proprietary reconstruction algorithm uses the landmarks to fit subregions of the right ventricle to various hearts in a catalog of patients with similar pathology. The algorithm then generates a 3D model on the basis of all the subregions. KBR is based on the piecewise smooth subdivision surface (PSSS) method, which incorporates knowledge regarding the locations of sharp edges at the valve planes. We sought to evaluate a KBR algorithm developed specifically for patients with pulmonary hypertension, focusing on a population with pulmonary arterial hypertension (PAH), in whom RV size and systolic performance have important prognostic implications.
Methods
Study Design and Population
Patients with World Health Organization group I PAH were recruited prospectively from the pulmonary hypertension clinic at the University of Chicago for same-day TTE and CMR, the latter of which was used as a reference standard. Recruitment specifically focused on patients who had expressed interest in ongoing research or had participated in prior clinical trials. Patients with contraindications to noncontrast CMR, such as inability to comply with breath-hold instructions, severe claustrophobia, or implanted ferromagnetic devices, were excluded. Patients were not screened for 2D transthoracic echocardiographic image quality before inclusion. CMR and transthoracic echocardiographic image acquisitions were separated by no more than 2 hours to minimize potential changes in RV size and function. On the same day, blood was drawn for N-terminal pro–brain natriuretic peptide (NT-proBNP) assessment, and 6-min walk testing was performed. The University of Chicago Institutional Review Board approved the study protocol. All patients provided written informed consent before study enrollment.
Echocardiographic Equipment, Image Acquisition, and Analysis
Transthoracic echocardiographic images were acquired using standard ultrasound equipment (iE33 system and S5 transducer; Philips Medical Systems, Andover, MA) connected to a specialized console by a transducer attachment and used with a magnetic field generator, located underneath the patient’s bed (VentriPoint Diagnostics Ltd., Seattle, WA). Before each study, the optimal ultrasound depth for the visualization of all relevant structures was determined and preset in the specialized console. Patients remained entirely stationary in the left lateral decubitus position throughout the imaging protocol. A series of standard and nonstandard transthoracic echocardiographic views were obtained, including the following: parasternal long-axis, parasternal short-axis at the papillary muscle level and at the apex, RV inflow, RV inflow-outflow, standard apical four-chamber, and focused RV apical ( Figure 1 ). Each acquisition consisted of two or three beats, and all images were acquired during breath holds. End-inspiratory acquisitions were preferred, but for patients with severe dyspnea, end-expiratory breath holds were permitted. All images were acquired by two sonographers (L.W. and M.Y.) trained in equipment use and image analysis.

Image analysis was performed on the specialized console. End-diastole was selected manually for each acquisition by visual determination of the time point at which RV volume was largest. End-systole was selected on an apical four-chamber view. The same end-diastole–to–end-systole interval was automatically applied to all other acquisitions. Points corresponding to several anatomic landmarks were placed on transthoracic echocardiographic images at end-diastole ( Table 1 ), with a minimum of nine points per patient. RV endocardial points were placed at the base of trabeculations. End-diastolic images were then submitted to a secure server and processed within seconds by a proprietary algorithm, KBR (VentriPoint Diagnostics Ltd.), created from a catalog of CMR and 2D transthoracic echocardiographic images from >100 patients with pulmonary hypertension. A 3D model of the right ventricle at end-diastole was then displayed for review on the console ( Figure 2 ). Transthoracic echocardiographic images with superimposed outlines of the 3D model were then reviewed to assess alignment ( Figure 3 ). Points were added and deleted as needed, and the images were reprocessed to optimize border alignment. On transthoracic echocardiographic images with obvious border misalignment, suggesting shifts in patient position, all points were deleted, effectively excluding these images from the model ( Figure 3 ). The same process of point placement, image processing, and model editing was performed at end-systole. Finally, a nested view of the end-diastolic and end-systolic models was reviewed to confirm appropriate alignment of tricuspid and pulmonic annular planes ( Figure 4 ). All transthoracic echocardiographic studies were analyzed by a single observer (N.M.B.) with formal training in software use, who was blinded to CMR results.
Site | Points |
---|---|
RV endocardium | 2 |
RV side of interventricular septum | 1 |
Tricuspid annulus | 3 |
Conal septum | 1 |
Pulmonic annulus | 1 |
Apex | 1 |
Basal bulge | 0 ∗ |
Subtricuspid RV endocardium | 0 ∗ |
RV septal edge | 0 ∗ |



CMR Image Acquisition and Analysis
CMR images were acquired using a 1.5-T scanner (Achieva; Philips Medical Systems, Best, The Netherlands) and a five-element phased-array cardiac coil. Retrospectively gated cine images were obtained with a steady-state free precession sequence (repetition time, 2.9 msec; echo time, 1.5 msec; flip angle, 60°; temporal resolution, ∼40 msec). A stack of short-axis slices (8-mm thickness, 2-mm gap) from the base to the apex of the entire heart was acquired. Short-axis cine slices were used to measure ventricular volumes and EFs using Simpson’s method of disks. All measurements were made by a single observer (see Acknowledgments), who was blinded to echocardiographic results.
Interobserver and Intraobserver Variability
All transthoracic echocardiographic studies were reanalyzed by a second observer (L.W.), who used the same end-diastolic and end-systolic frames and excluded the same misaligned images as the original observer. The second observer was blinded to the original observer’s end-diastolic volume (EDV), end-systolic volume (ESV), and EF measurements. For intraobserver variability assessment, the original observer (N.M.B.) performed repeat analyses ≥24 hours after the initial analyses. Interobserver and intraobserver variability was quantified using intraclass correlation coefficients and coefficients of variation, the latter of which were defined as the absolute difference between repeated measurements as a percentage of their mean.
Statistical Analysis
The relationship between TTE-derived and CMR-derived RV volumes and EFs was evaluated using linear regression analysis with Pearson’s correlation coefficient. In addition, Bland-Altman analysis was performed to assess agreement between the two imaging modalities in terms of bias and 95% limits of agreement.
Results
From November 2011 to October 2012, 29 patients were recruited and agreed to participate in the study. Two patients were excluded: one because of changes in position during TTE that resulted in severe image misalignment and one because of refusal to undergo CMR. Approximately 10 additional patients were approached but declined to participate. Twenty-seven patients (96% women) were included in the final analysis. Patient characteristics are displayed in Table 2 . The mean number of acquisitions performed per study was 15.5 ± 2.4 (range, 11–20), generally requiring 10 to 15 min of scanning time. Image quality was sufficient to allow point selection and reconstruction in all included patients. Image analysis generally required 15 to 20 min per patient, with two to five reconstructions each at end-diastole and end-systole. End-diastolic models contained a mean 39 ± 11 points (range, 18–64) and end-systolic models 37 ± 11 points (range, 20–58).
Variable | Value |
---|---|
Age (y) | 52 ± 14 |
Women | 26 (96%) |
Caucasian | 16 (59%) |
Etiology of PAH | |
Idiopathic | 10 (37%) |
Connective tissue disease | 7 (26%) |
Anorexigen | 5 (18%) |
Congenital heart disease | 4 (15%) |
HIV | 1 (4%) |
WHO functional class | |
I | 8 (30%) |
II | 13 (48%) |
III | 6 (22%) |
Years since PAH diagnosis | 6.7 ± 5.8 |
PAH medications | |
Prostacyclin | 18 (67%) |
PDE-5 inhibitor | 19 (70%) |
Endothelin receptor antagonist | 6 (22%) |
Calcium channel blocker | 2 (7%) |
Home oxygen | 13 (48%) |
Body surface area (m 2 ) | 1.8 ± 0.3 |
Heart rate (beats/min) | 79 ± 13 |
Systolic blood pressure (mm Hg) | 120 ± 20 |
Diastolic blood pressure (mm Hg) | 69 ± 14 |
NT-proBNP (pg/mL) | 1,113 ± 1,537 |
6-min walk distance (m) | 380 ± 105 |
RV EDV (mL) | 230 ± 67 |
RV ESV (mL) | 157 ± 59 |
RV EF (%) | 33 ± 10 |
SV (mL) | 73 ± 22 |
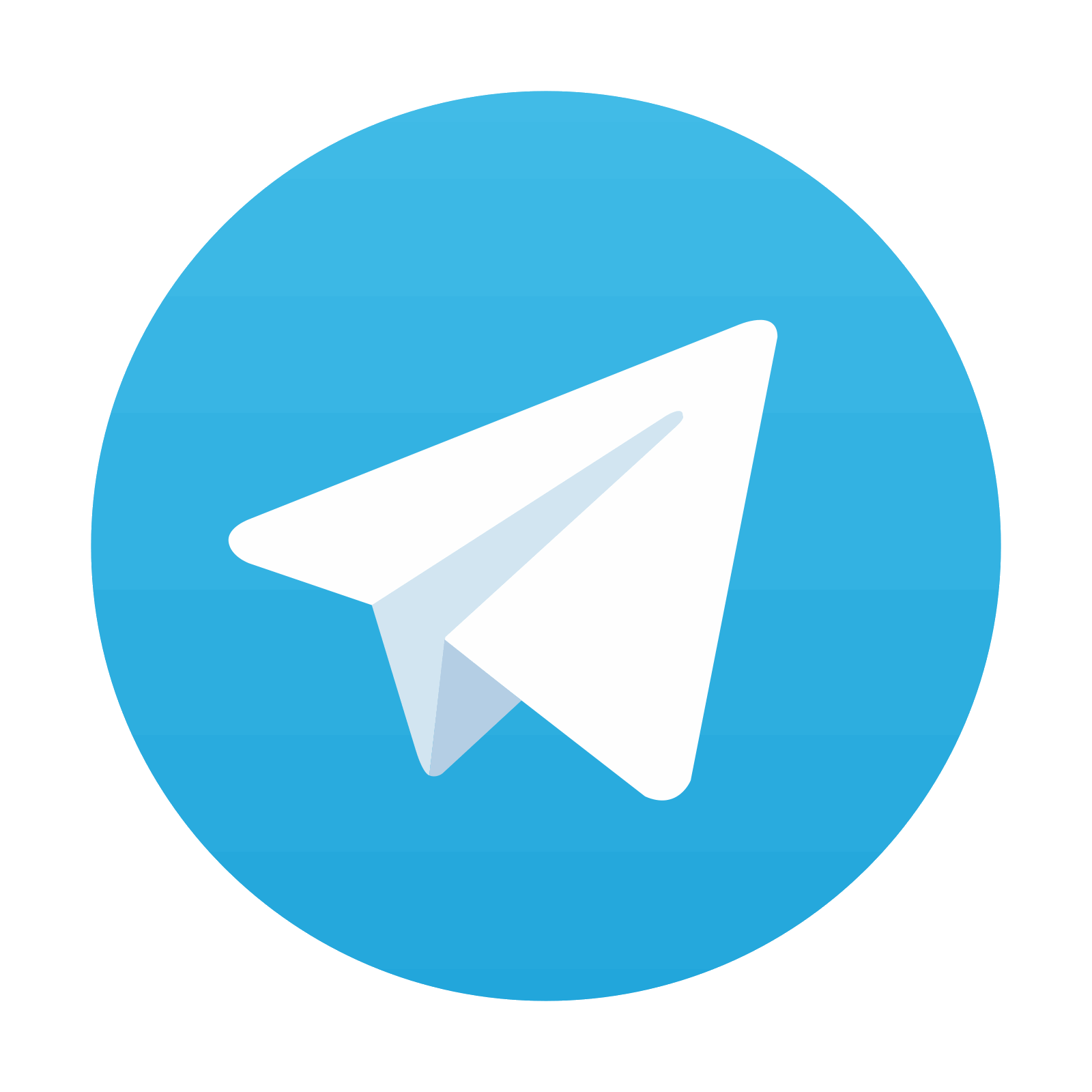
Stay updated, free articles. Join our Telegram channel
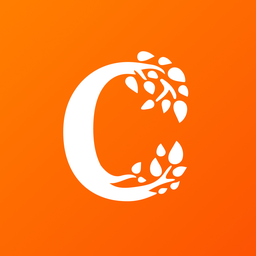
Full access? Get Clinical Tree
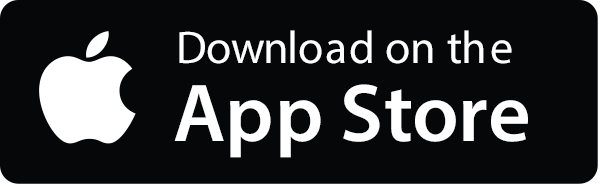
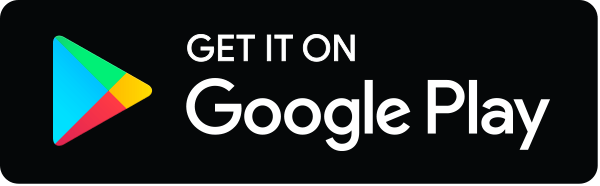
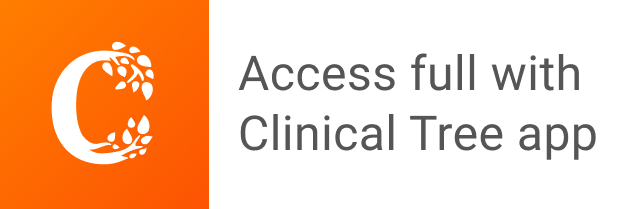