Appreciation of complex intracardiac anatomy and spatial relationships is inherent to the diagnosis of congenitally malformed hearts. Beginning over 30 years ago, and until recently, the ability of the clinician to image the heart by echocardiography was limited to cross sectional techniques displayed in two dimensions. 1 Such two-dimensional echocardiography has fundamental limitations. The very nature of a two-dimensional slice, which has no thickness, necessitates the use of multiple orthogonal sweeps. The echocardiographer then mentally reconstructs the anatomy, and uses the structure of the report to express this mentally reconstructed vision. This means that the only three-dimensional image of the heart available for diagnosis is the virtual image existing in the mind of the echocardiographer, who then translates this vision into words when preparing the report. It is not easy for an untrained, albeit interested, observer to understand the images obtained in the course of a sweep. Expert interpretation is required. Furthermore, since myocardial motion occurs in three dimensions, two-dimensional echocardiographic techniques do not lend themselves to accurate quantitation.
Recognition of these limitations of cross sectional echocardiography seen in two dimensions led to burgeoning research and clinical interest in three-dimensional echocardiography. Early reconstructive approaches were based on acquisitions of large numbers of two-dimensional images that were subsequently stacked and aligned based on the phases of the cardiac cycle, in this way producing a three-dimensional dataset. 2–4 While these approaches proved to be accurate, the need for time and offline processing equipment imposed fundamental limitations on their clinical applicability. In 1990, von Ramm and Smith published their early results with a matrix-array transducer that provided real-time images of the heart in three dimensions. 5 While this was an important breakthrough, this transducer was unable to be steered in the third dimension, which has since been termed the plane of elevation. Over the past 5 years, dramatic technological advances have facilitated the ability to perform live three-dimensional scanning, including the ability to steer the beam in three dimensions, and to render the image in real time. 6
ADVANCES IN TECHNOLOGY
Contemporary transducers based on arrangement of elements in a matrix, piezoelectric materials, and quantitative software are the three elements that have facilitated enhancements in three-dimensional echocardiography.
Matrix-array Transducers
These transducers comprise as many elements in the elevation dimension as they do in the azimuth dimension, with more than 60 elements in each. While the elements are arranged in a two-dimensional grid, this array generates three-dimensional echocardiographic images. In order to be able to steer in the elevation plane, each element must be electrically independent from all other elements, and each element must be electrically active. The technology and electrical circuitry to insulate electrically, and connect each element, became commercially available in 2002. As a result, these transducers consist of thousands of electrically active elements, each of which independently steers a scan line left and right, as well as up and down.
Piezoelectrical Materials
The piezoelectrical material in a transducer fundamentally determines the quality of the image. Piezoelectrical elements are responsible for delivery of ultrasonic energy into the tissue that is scanned, and for converting waves of reflected ultrasound into electric signals. Their efficiency in converting electrical energy to mechanical energy, and vice versa, is a key determinant of the quality of the image, sensitivity to Doppler shifts, and the ability of transmitted ultrasound to penetrate to increasing depths. In order to create a piezoelectrical effect, these elements must be subjected to the application of an external electric field to align dipoles within polycrystalline materials. For almost 40 years, a ceramic polycrystalline material named lead-zirconate-titanate has been the piezoelectrical material that has enjoyed standard usage in medical applications. This material is a powder that is mixed with an organic binder. The resulting compound is baked into a dense polycrystalline structure. At its best, it achieves approximately 70% alignment of dipoles. This inherently constrains the efficiency of the material to achieve electromechanical coupling.
One example of new piezoelectrical material involves growing crystals from molten ceramic material, resulting in a homogenous crystal with fewer defects, lower losses, and no boundaries between grains. 7,8 When these crystals are poled at the preferred orientation or orientations, a near-perfect alignment of dipoles is achievable, resulting in dramatically enhanced electromechanical properties. The efficiency of conversion of electrical to mechanical energy improves by as much as two-thirds to five-sixths when compared to lead-zirconate-titanate ceramics, which are otherwise currently used in ultrasonic transducers. The new piezoelectrical materials provide increased bandwidth and sensitivity, resulting in improvements in both penetration and resolution. The improved arrangement of atoms in these new piezoelectrical materials, and their superior strain energy density, has translated into advances in miniaturisation. These advances led to the availability of a high-frequency matrix three-dimensional transthoracic transducer that has dramatically enhanced the applicability of three-dimensional echocardiography for use in children. 9 Another example of emerging transducer materials consists of silicon elements. This technology is being developed at the present time.
Software for Quantification
Quantification requires that the software provide the ability to separate out and segment structures of interest from the acquired data. Two-dimensional quantitative techniques are based on geometric formulas that rely on assumptions regarding the shapes of cardiac structures. These assumptions are frequently incorrect. In contrast, three-dimensional acquisitions include the entire extent of the structure, thus minimizing the possibility of foreshortening of the apex or any geometric assumptions regarding shape. Three-dimensional quantitative software has the potential to quantify cardiac structures accurately regardless of their shape. Advances in software for processing three-dimensional data sets have mirrored the rapid advances in transducer technology that have occurred over the past few years.
Three-dimensional volumetric techniques rely on definition of chamber cavities, that is, the interface between blood and endocardium. The software constructs this interface by using a process known as surface rendering, and represents it as a mesh of points and lines. This software-generated mesh is calculated for every frame of acquisition, thus providing a moving cast of the cavity of the ventricle during the cardiac cycle. Since this is digital data, it is easy to compute global and regional volumes, synchrony as well as parametric displays of endocardial excursion, and timing of contraction ( Fig. 18B-1 ).

Tools for three-dimensional quantification of the left ventricle are more technologically advanced than for other cardiac structures. Until recently, such tools employed the method of disk summation. With improvements in computing speeds and programming, newer tools have been developed to provide instantaneous tracking of the interface between the blood pool and endocardium at each frame of acquisition. This provides a surface-rendered model that is displayed as a mesh of lines and points. These algorithms, nonetheless, are still based on some basic three-dimensional geometric assumptions regarding left ventricular shape, and therefore they cannot be applied to the right ventricle or to functionally univentricular hearts. Given the complex shape and architecture of the right ventricle, it is unsurprising that tools for quantifying its volume have been slower to mature. Until very recently, these tools utilised the method of disks for volumetrics. 10 Novel software now provides the ability instantaneously to track the interface of blood pool and endocardium at each frame of acquisition ( Fig. 18B-2 ), yielding a surface-rendered model that is displayed as a mesh of lines and points. 11

In a recent development, quantitative software for the mitral valve has been developed. This provides the ability to perform sophisticated analyses of the nonplanar shape of the mitral valvar annulus, and to measure dimensions including annular diameters, lengths of the zone of apposition between the leaflets, and the surface areas of each leaflet. 12,13 Quantitative techniques have also been developed to provide volumetric measurements of three-dimensional colour flows using non-aliased data. 14
CLINICAL APPLICATIONS IN PATIENTS WITH CONGENITALLY MALFORMED HEARTS
Three-dimensional echocardiographic imaging has three broad areas of clinical application among patients with congenitally malformed hearts, namely, the visualisation of morphology, the quantification of sizes of chambers and flows of blood, and the emerging area of image-guided interventions.
Visualizing Morphology
Dating from an early stage in the development of three-dimensional technology, the structural complexity that is inherent to the congenitally malformed heart has been identified as fertile substrate for exploration using three-dimensional echocardiography. 15–17
Atrioventricular Valves
Three-dimensional echocardiography is valuable in delineating the morphology of the atrioventricular valves, with the technique being used at an early stage to delineate congenital abnormalities of the mitral valve, 18 including comprehensive assessment of double orifices. 19 The additive value of the technique, and improved quality of images, was then demonstrated in the intra-operative environment. 20 More recently, the technique was shown to be capable of delineating the morphology of the leaflets, cordal attachments, the subcordal apparatus, the mechanism and origin of regurgitation, and the geometry of the regurgitant volume. 21 In the setting of Ebstein’s malformation of the tricuspid valve, the technique was shown to provide clear visualisation of the morphology of the valvar leaflets, including the extent of their formation, the level of their attachment, and their degree of coaptation. 22
Atrioventricular Septal Defect
In patients with atrioventricular septal defect, we have found that three-dimensional echocardiographic imaging provides unparalleled views of the zone of apposition between the superior and the inferior bridging leaflets of the left component of the common atrioventricular valve ( Fig. 18B-3 ). Our experience with a cohort of patients with atrioventricular septal defects 23 showed that gated three-dimensional echocardiographic views could be cropped to reveal the relationships of the bridging leaflets to the septal structures ( Figs. 18B-4 and 18B-5 ). These views have been useful in determining the precise level of shunting, particularly prior to surgical repair. The technique was of particular value in patients with unbalanced defects who were being considered for biventricular repair.



Atrial and Ventricular Septal Defects
Others have shown that three-dimensional echocardiography provides unique views of the surfaces of the atrial and ventricular septal structures, revealing the holes between adjacent chambers. 24,25 The technique is of particular value in demonstrating the morphology of defects within the muscular ventricular septum, 26 and in assessing malformations of the outflow tract involving malalignment of the outlet septum.
Aortic Arch, Pulmonary Arteries, and Aortopulmonary Shunts
We have shown ( Fig. 18B-6 ) the value of colour flow Doppler in providing echocardiographic angiograms of the patterns of flow in the obstructed aortic arch, the right and left pulmonary arteries subsequent to the Lecompte maneuver, and across Blalock-Taussig shunts. 27 In this recent experience, validation of the diagnosis made by three-dimensional echocardiography was confirmed at surgery, by cardiac catheterisation, or by magnetic resonance or computerised tomographic imaging in two-thirds of the patients.
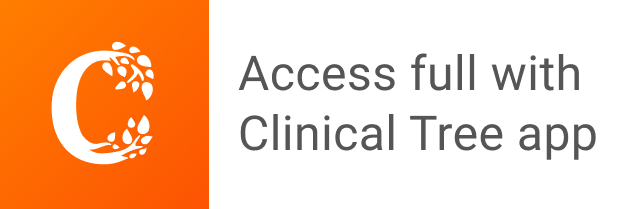