Background
The modern era of extracorporeal circulation began in the early 1950s, with cardiopulmonary bypass (CPB) used to support patients during open-heart operations for the repair of congenital heart defects. Over the course of the next two decades, improvements to the CPB circuits and oxygenator technology, especially the advent of the membrane oxygenator in the early 1970s, led to the development of extracorporeal membrane oxygenation (ECMO). This new technology allowed for efficient shunting of blood between the venous and arterial systems while actively oxygenating the blood, thus providing cardiac and respiratory support. While the original CPB technology achieved the same goal, the advent of the membrane oxygenator allowed this technology to be used in the intensive care unit. As extracorporeal circulatory support technology continues to improve and evolve ( Fig. 6.1 ), its use is more prevalent than ever.

The first report of successful ECMO support was in 1972 in an adult patient with advanced respiratory failure as a consequence of severe pulmonary contusion. This success resulted in a fervor of enthusiasm for the therapy, which was abruptly stunted 2 years later when a clinical trial only had 2 survivors out of 17 patients supported with ECMO. In the decades that followed, ECMO was rarely utilized in adults, but ECMO use was rapidly expanded to pediatric patients with congenital heart disease, meconium aspiration, or diaphragmatic hernia when no other options were available. As a therapy for adult patients, ECMO use was limited to acute cardiogenic shock refractory to medical therapy or to patients with inability to come off CPB after complex cardiac operations. Limitations of the pump and oxygenator technology limited prolonged and stable support. Adults who were supported with ECMO had high rates of associated complications, including bleeding, thromboembolism, early oxygenator failure, disseminated intravascular coagulopathy, and systemic inflammatory response syndrome. These complications, coupled with the availability of other circulatory support options, limited the use of ECMO, such that it was considered only occasionally as a salvage option in patients with very advanced cardiopulmonary failure.
Fortunately, with the development of polymethylpentene (PMP) oxygenators, bearing-less centrifugal pumps, and better cannula technology, there has been a rapid increase in the use of ECMO for cardiac support since 2009 ( Fig. 6.2 ). ECMO has been successfully used as a bridge to cardiac recovery, a bridge to heart transplantation (BTT), a bridge to a more permanent left ventricular assist device (LVAD), a or as bridge-to-bridge (BTB). The indications for adult ECMO have also expanded to include patients with various etiologies of cardiac failure, including acute myocardial infarction (AMI), end-stage dilated cardiomyopathy, refractory ventricular arrhythmias, acute myocarditis, the inability to wean from bypass after cardiac surgery, and cardiac arrest. The ability of ECMO to provide complete cardiac support (left and right ventricle) as well as oxygenation has several advantages over some other percutaneous and surgically implanted temporary devices. Its ease of implantation and versatility have made ECMO the most widely used circulatory support system, with close to 2500 implants per year in the United States for cardiac support ( Fig. 6.2 ).

Current ecmo technologies used in cardiac support
ECMO combines the use of a pump (ideally a centrifugal pump) for blood propulsion and a membrane oxygenator for gas exchange. Other components include the pump electrical drive unit (controller), tubing, cannulas, and a heat exchanger to prevent temperature loss ( Fig. 6.3 ). A venous cannula is used to drain deoxygenated blood into the pump. This pump then pushes the blood through a membrane oxygenator for gas exchange, and then the oxygenated blood is returned to the patient via an arterial cannula, resulting in complete respiratory and hemodynamic support. The amount of blood flow through the circuit and the fraction of oxygen delivered through the oxygenator are the main determinants of blood oxygenation and circulatory support. The rate of gas flow through the oxygenator, known as the sweep gas flow rate, and the blood flow rate are the major determinants of carbon dioxide removal. In other words, as the flow of sweep gas through the oxygenator increases relative to the flow of blood through the oxygenator, the CO 2 content of the blood leaving the oxygenator and returning to the body decreases. Currently, there are no US Food and Drug Administration–approved devices for ECMO. The current systems have all been approved through the 510K device approval pathway for a 6-hour extracorporeal life support indication. However, in practice, the use of this technology has been extended far beyond those limits, with an average cardiac ECMO support time of 166 hours as per the 2017 Extracorporeal Life Support Organization (ELSO) annual report.

Initially, roller pumps were used for blood propulsion in ECMO circuits. These pumps work by squeezing tubing carrying the blood to cause forward flow; with prolonged use, they result in significant blood trauma. Centrifugal pumps, on the other hand, have theoretically less associated blood trauma and have essentially replaced roller pumps in adult ECMO. The use of centrifugal pumps has been frequently associated with significantly decreased blood trauma, thrombus formation, secondary hemolysis, and acute pump failure, although some controversy still exists. Centrifugal pumps are preload dependent, meaning that their flow rates are sensitive to the volume available to the pump inlet. They generate a negative inlet pressure, allowing for kinetic drainage. Centrifugal pumps are also after load sensitive, meaning that their flow rates are inversely responsive to the pressure at the pump outlet. For this reason, if the patient’s blood pressure is abnormally elevated, ECMO flow decreases. This is a significant advantage relative to roller pumps because kinks in the tubing after the centrifugal pump can result in decreased flow, but not the catastrophic tubing ruptures that have happened with roller pumps. These properties also allow the centrifugal pump to be closer to the patient resulting in shorter lines, smaller priming volume, decreased surface area of blood exposure, and decreased blood transit time.
First-generation centrifugal pumps had a constrained vortex design with a closed center, a mechanical bearing, and a direct coupling of the pump rotor to drive motor. This design resulted in significant blood stagnation potential at various locations within the pump. To combat this stagnation potential, second-generation pumps used an impeller with an open-center “Mendler” design. Additionally, these pumps are magnetically coupled to the drive motor and use a pivot bearing. Third-generation pumps share similar bloodflow design, but with a “bearing-less” fully magnetically levitated impeller. This decreases heat generation and the likelihood of pump thrombosis. The pumps most frequently used for ECMO support in the United States include the Maquet Rotaflow (Getinge), the Sorin Revolution (LivaNova), and the St Jude Centrimag (Abbott) ( Fig. 6.4 ). Newer centrifugal pump technologies (which are not approved for use in the United States) are commercially available in the European Union with CE Mark approval, including the Medos Deltastream DP3, which boasts minimal priming volume and the ability for pulsatile flow.

Improvements in oxygenator technology have been even more important than pump improvements for the recent success of ECMO support in treating cardiopulmonary disease. The advent of membrane oxygenation made ECMO possible, because all previous oxygenator technologies caused too much blood trauma. The first membrane oxygenators used solid silicone membranes for oxygen exchange. These oxygenators were limited by a high rate of platelet deposition and thrombus formation that affected the durability and functionality of the oxygenators and resulted in early failure. Subsequently, the development of microporous, hollow-fiber (polypropylene) membranes allowed for better oxygen transfer with decreased membrane oxygenator size. Since the mid-2000s, the use of nanoporous hollow fibers with PMP has revolutionized oxygenator technology, allowing for prolonged support with decreased blood component trauma, decreased platelet activation, lower thromboembolism, and decreased device-related bleeding complications. These PMP membranes have a compressed (skinned) surface, reduced pore size (< 0.03 μm), high gas permeability, and infrequent plasma leakage. The PMP oxygenators most often used in the United States are the Quadrox D (Maquet/Getinge) and the EOS ECMO (Sorin/LivaNova) ( Fig. 6.5 ), but the HiLiteLT (Xenios) and Novalung (Xenios) have also been available. Additionally, the newest oxygenators have integrated connections for heat exchange, which facilitate patient temperature control. More recently, integrated ECMO systems have been developed that couple a second-generation centrifugal pump with a PMP oxygenator, allowing for a decrease in the overall ECMO system size and increased portability. In general, while the “a la carte” combination of second- and third-generation pumps with PMP nanoporous oxygenators has been quite successful (Rotaflow/Centrimag pumps and Quadrox D oxygenator or Sorin Revolution or EOS Oxygenator), the use of integrated systems (Maquet CardioHelp or Tandem Lung) has become increasingly popular due to decreased size and improved portability ( Fig. 6.6 ). Despite a multitude of available ECMO systems, there is no scientific evidence supporting the superiority of any of the modern technologies in patients supported with either venoarterial (VA) or venovenous (VV) ECMO.


Unlike pumps and oxygenators, there has been less innovation to cannula technologies for cardiac support. The current cannula designs have been borrowed from peripheral CPB systems. They are tapered, which facilitates peripheral (percutaneous or direct) cannulation as well as direct central cannulation, but they lack modifications for long-term use. While the cannulas have excellent flow characteristics (many achieve up to 6 L/min with minimal pressure gradient), they lack innovations that facilitate distal perfusion of peripheral vessels or enable easy percutaneous removal. Most of the innovation in tubing and cannula design has been focused on improving biocompatibility. Most tubing, cannulas, and other circuit components have a proprietary biocompatible surface coating (heparin, phosphorylcholine, poly-2-methoxy-ethyl acrylate (PMEA), and others) to decrease blood component activation, platelet deposition, and thrombus formation.
While there have been significant advancements in ECMO technology over the years, there is still significant room for improvement. ECMO system designs currently under development and in the early phases of clinical use may allow further decreases in pump and oxygenator size, improved portability, and even the ability to provide counter pulsation through pump speed changes. Ultimately, the goal of any innovation in ECMO technology will be to improve biocompatibility, safety, and clinical outcomes.
Ecmo configurations and cannulation strategies for cardiac support
ECMO can be configured for either primarily respiratory support, VV-ECMO, or circulatory support, VA-ECMO. In VV-ECMO, deoxygenated blood is withdrawn from the venous system and oxygenated blood is returned to the venous system, effectively scrubbing CO 2 and preoxygenating blood before it is pumped to the lungs by the heart. During VA-ECMO, deoxygenated blood is withdrawn from the venous system and oxygenated blood is returned to the arterial system providing circulatory support. Occasionally, a hybrid technique where oxygenated blood is returned to the venous system and the arterial system simultaneously (known as veno-arterio-venous [VAV] or veno-veno-arterial [VVA] ECMO) is used, usually in patients with severely decreased pulmonary function or significant pulmonary shunt.
Cannulation techniques for VA-ECMO depend on the clinical condition of the patient and the available vascular access. In patients with nonoperative cardiogenic shock (e.g., AMI, myocarditis, and decompensated chronic heart failure), percutaneous peripheral cannulation of the femoral artery and femoral vein is the most frequently used technique in adults ( Fig. 6.7 A ). In the presence of femoral-iliac vascular disease, peripheral open subclavian artery cannulation is a useful option. This technique requires the creation of an end-to-side “chimney graft” with a Dacron graft to prevent subclavian arterial damage (see Fig. 6.7 B). In these patients, the venous drainage cannulation can be placed in the femoral vein or the internal jugular (IJ) vein. Patients who have shock during or after cardiac surgery (through a sternotomy) can be supported with central cannulation, typically achieved with direct cannulation of the right atrium and aorta ( Fig. 6.8 ).


For percutaneous femoral cannulation, the femoral artery is usually located by ultrasound and accessed using the modified Seldinger technique. After progressive arterial dilation is performed using tapered dilators, the arterial cannula is then inserted over the guidewire and its tip is positioned in the distal abdominal aorta or proximal common iliac artery. Peripheral arterial cannulas sized 15–16 Fr are used for smaller patients (< 60 kg), and 17–21 Fr cannulas are used for larger patients. A similar technique is used for the cannulation of the femoral vein, with the goal of placing the cannula tip in the body of the right atrium. When patients are cannulated emergently, arterial and venous cannulation occurs on whichever side is accessed first. In patients in more stable condition, the authors prefer to cannulate the right femoral vein and the contralateral femoral artery. This theoretically facilitates venous return in the leg with arterial cannulation and decreases the risk of limb ischemia. In the absence of image guidance (fluoroscopy or transesophageal echocardiography), secondary signs of wire placement must be used for venous cannulation, including ventricular ectopy and the “feel” of the wire. Generally, adequate venous drainage can be achieved with a 22 Fr multi-fenestrated venous cannula in smaller patients (< 60 kg) and a 25 Fr multi-fenestrated venous cannula in larger patients; the target ECMO flow rate is 2.0–2.2 LPM/m 2 . When ECMO cannulation is placed during cardiopulmonary resuscitation (CPR) in patients with small femoral vessels or patients whose vessels cannot be easily located on ultrasound, an open technique can be utilized. After the vessels are exposed surgically, cannulation is performed either directly through a purse string, over the wire through a purse string (“semi-Seldinger”), or through an end-to-side Dacron graft sewn to the artery.
Femoral arterial cannulation can jeopardize perfusion of the distal femoral artery and can be abated with a distal perfusion catheter ( Fig. 6.7 A). The use of distal arterial perfusion catheters is recommended in all patients undergoing peripheral VA-ECMO because there is increasing evidence that distal perfusion decreases the rate of serious vascular complications. If the patient is relatively stable, inserting the distal perfusion cannula prior to femoral arterial cannulation is preferred; this facilitates identification of the distal superficial femoral artery. Then, using the modified Seldinger technique, a 5–10 Fr cannula or sheath is introduced in the superficial femoral artery and connected to the arterial limb of the circuit ( Fig. 6.9 ). It is important to confirm the passage of the wire with ease into the distal part of the artery as the guide wire and cannula can easily be positioned in the profunda femoris artery, leading to subsequent ischemia of the lower extremity. If the procedure is performed in the operating room or if there is a specific concern regarding distal lower extremity perfusion, angiography should be performed to confirm proper positioning. Some clinicians routinely confirm positioning using angiography. There is recent evidence that near-infrared spectroscopy may identify limb ischemia, and advocates recommend monitoring both limbs for the duration of ECMO support as ischemia may occur in up to 30% of ECMO patients.

Peripheral VA-ECMO support will increase mean arterial pressure and, in turn, increase left ventricular (LV) afterload. In some patients, this may cause an increase in LV end-diastolic pressure, leading to pulmonary injury and severe pulmonary congestion/edema. The resulting pulmonary shunt will fill the LV with poorly oxygenated blood that may be ejected every cardiac cycle. If patients are femorally cannulated, this deoxygenated blood can fill the root of the aorta and head vessels, resulting in central hypoxia, known as North-South syndrome ( Fig. 6.10 ). If the ventricle does not eject while on VA-ECMO, there can be intraventricular, LV outflow tract, or sinus of Valsalva thrombus formation, as well as severe LV distention, resulting in coronary ischemia, arrhythmias, or cerebrovascular thromboembolism. Animal studies have demonstrated that peripheral VA-ECMO can cause pressure-volume curve dissociation, decreased coronary perfusion, and poor LV unloading when compared with devices that directly drain the LV. To minimize the risks associated with femoral ECMO, patients should be monitored closely, and proactive steps should be taken to prevent complications. Specifically, it is important to prevent LV distention, LV thrombosis, and lung injury. This can be achieved either by placing an axial blood pump across the aortic valve to decompress the LV or by direct venting of the LV with a cannula placed either in the LV apex or in the right superior pulmonary vein, diverting blood into the venous limb of the ECMO circuit. LV unloading has been associated with improved survival and decreased pulmonary congestion and may facilitate myocardial recovery.

In patients with North-South syndrome, VAV-ECMO ( Fig. 6.11 ) has been used to improve oxygenation of blood that is ejected by the LV. This is achieved with the placement of a second cannula (16–18 Fr) in the right IJ vein. The insertion of this IJ cannula is similar to femoral venous cannulation using the modified Seldinger technique. The cannula is connected to the arterial limb of the ECMO circuit, in addition to the existing cannula in the arterial system. Oxygenated blood returns to the patient from both the femoral artery, providing circulatory support), and the right IJ vein, providing pulmonary support. Because of the compliance difference between the venous and arterial vascular trees, it is important to monitor and regulate flow between these “oxygenated” limbs. Flow is monitored using an ultrasonic flow probe and can be diverted from the right IJ cannula to the femoral cannula by partially occluding the right IJ cannula using a Hoffman clamp.

The most definitive technique for preventing LV thrombus formation is venting the LV, either with a surgically placed cannula or with a transvalvular axial pump. In the absence of an LV vent, maintaining contractility to allow LV ejection and adequate anticoagulation will minimize the risk of thrombus formation. Typically, partial thromboplastin time is maintained at 50–70 seconds with intravenous heparin, if there is no concern for bleeding. LV ejection can be maintained by providing inotropic medications, reducing afterload (with medicines or an intra-aortic balloon pump), and optimizing volume status. A recent study compared the use of ECMO vs. ECMO and Impella for LV drainage. The findings suggested a survival benefit and improved ability to wean ECMO in patients whose LV was unloaded, suggesting that active venting may be beneficial.
VA-ECMO using central cannulation is used frequently in patients presenting with cardiogenic shock after cardiac operations or in cases where peripheral access is not an option (e.g., femoral-iliac occlusive disease). Due to the antegrade flow in the aorta, central VA-ECMO is generally not associated with North-South syndrome and, may provide better unloading of the left and right ventricles than peripheral femoral ECMO, due to the larger drainage cannulas. After exposure of the heart via sternotomy or right anterior thoracotomy, purse-string sutures are placed in the ascending aorta and right atrium; the sutures are captured with tourniquets. Although standard CPB cannulas can be used for central ECMO, the authors prefer to use tapered percutaneous cannulas. This allows for exteriorization through the abdominal wall ( Fig. 6.8 ) and, potentially, complete closure of the chest. For venous drainage, typically a large venous cannula (> 28 Fr) is selected. Once on support, the LV is evaluated for distention and stasis. If there is any concern, a surgical vent is placed using purse-string sutures in the pulmonary artery, in the right superior pulmonary vein, or at the LV apex. Typically, temporary closure of the sternotomy is achieved by approximating the skin with a continuous suture without approximation of the sternal bone. If the skin cannot be approximated, an Esmarch bandage can be used. It is advisable to only temporarily close the median sternotomy to facilitate reexploration for postoperative bleeding, which is common.
Characteristics of ecmo vs other temporary mechanical circulatory support systems
In addition to ECMO, there are an increasing number of options for percutaneous circulatory support ( Table 6.1 ). Catheter- or cannula-based vascular access with mechanical pumps can be used as first-line rescue therapy in patients with refractory cardiogenic shock (e.g., percutaneous LVADs). Additionally, surgically implanted temporary ventricular assist devices (VAD) (e.g., Centrimag) have been used for many years in this patient population. While VA-ECMO is still the only percutaneous circulatory support that can be implanted emergently at the bedside, there are now other options for percutaneous left, right, and biventricular support. Most notably, percutaneous right ventricular support can be provided with the Impella RP device (AbioMed) or with the ProTek Duo cannula (LivaNova) coupled with a centrifugal pump. These devices can be used in combination with Impella devices (2.5, CP, 5.0, LD) for LV support or the Tandem Heart LVAD to provide percutaneous biventricular support. Most commonly, these devices are used along with ECMO support to stabilize a patient waiting for recovery or definitive therapy.
Characteristic | Impella 2.5–3.5 CP/RP | Impella 5.0/5.5 | TandemHeart LVAD–RVAD | ECMO | Centrimag Temporary VAD |
---|---|---|---|---|---|
Bedside implantation | No | No | No | Yes (peripheral ECMO) | No |
Flow L/min | 2.5–3.5 | 4.5–5 | 3–4.5 | 3–6 | 4–6 |
LV unloading | Yes | Yes | Yes | Partial | Yes |
RV support | Yes | Yes | Yes | Yes | Yes |
Pulmonary support | No | No | No | Yes | no |
Duration of support | Days–weeks | Days–weeks | Days–weeks | Days–weeks | Weeks–months |
Insertion | Percutaneous | Graft | Percutaneous | Percutaneous/surgical | Surgical |
Cannula or catheter/pump size | 9 Fr/12–14 Fr 22 Fr RP | 9 Fr, 19–21 Fr | 17-21 Fr LVAD 29–31 Fr RVAD (Protek) | 15-17 Fr arterial 22–25 Fr ven. |
Indications for ecmo in patients with cardiac disease
Box 6.1 summarizes the most frequent indications for ECMO use in patients with cardiac disease and contraindications to VA-ECMO. The indications for ECMO for cardiac support fall broadly into the following categories:
- 1.
Cardiogenic shock refractory to multiple vasoactive medications or an existing mechanical circulatory support (MCS) device, such as an intraaortic balloon pump
- 2.
Cardiac arrest unresponsive to conventional CPR (extracorporeal CPR, commonly known as ECPR)
- 3.
Refractory ventricular arrhythmias
- 4.
Acute or decompensated right heart failure in the context of pulmonary vascular disease (e.g., pulmonary hypertension or pulmonary embolism)
Indications
- •
Myocardial infarction–associated cardiogenic shock
- •
Fulminant myocarditis
- •
Sepsis-associated cardiomyopathy
- •
Adult congenital heart disease with acute decompensated heart failure
- •
Postcardiotomy cardiogenic shock
- •
RV support during LVAD implantation
- •
Bridge to VAD or heart transplantation
- •
Posttransplantation graft failure
- •
ECPR
- •
Cardiogenic shock post–cardiac arrest
- •
Refractory ventricular arrhythmia
- •
Pulmonary hypertension with RV failure
- •
Massive pulmonary embolism
Contraindications
Absolute
- •
Severe irreversible noncardiac organ failure limiting survival (e.g., severe anoxic brain injury)
- •
Irreversible cardiac failure if transplantation or long-term VAD will not be considered
- •
Severe aortic insufficiency
- •
Aortic dissection
Relative
- •
Severe coagulopathy or contraindication to anticoagulation
- •
Limited vascular access
- •
Severe peripheral arterial disease
- •
Age > 75 years
ECMO , Extracorporeal membrane oxygenation; ECPR , extracorporeal cardiopulmonary resuscitation; LVAD , left ventricular assist device; RV , right ventricle; VAD , ventricular assist device.
Unfortunately, unlike studies on ECMO for respiratory failure, there is an absence of randomized controlled trials examining the efficacy of ECMO for the treatment of cardiogenic shock. The reports in the literature are limited to cohort studies, cases series, metaanalyses, and case reports.
Heart Failure and Cardiogenic Shock
There are a broad range of therapies for patients with decompensated acute or chronic heart failure who present in cardiogenic shock. These include intravenous inotropes, intraaortic balloon pumps, peripherally inserted VADs, surgically implanted short-term extracorporeal VADs, VA-ECMO, and durable VADs. Despite a paucity of data supporting ECMO as a superior therapy, the utilization of ECMO in patients with heart failure is increasing, likely due to the ease of ECMO implantation and its ability to provide biventricular support. Based on expert consensus, ECMO can be considered in patients with cardiogenic shock due to (1) myocarditis, (2) cardiomyopathy, (3) myocardial infarction, (4) refractory malignant arrhythmia, (5) pulmonary embolism, (6) pulmonary hypertensive crisis, or (7) posttransplantation graft dysfunction. While the indications for cardiac ECMO support are broad, the contraindications are consistent across etiologies. ECMO should be avoided in patients who have severe and irreversible neurological injury, irreversible cardiac failure without candidacy for transplant or LVAD placement, aortic dissection, severe aortic valve insufficiency, or any noncardiac comorbid condition that limits their life expectancy to less than 1 year.
Frequently, the call to consider a patient for ECMO support is done when the patient exhibits advanced stages of shock with limited time to define the most appropriate treatment option, and ECMO is generally considered as a bridge to decision. Once the clinical condition of the patient has been stabilized and the treatment course established using a multidisciplinary team approach, a destination therapy can be defined, including bridge to recovery, a durable VAD (BTB), or heart transplantation. If none of these options is viable due to progressive deterioration of multiorgan failure, preexisting conditions that preclude further therapy (e.g., advanced cancer), or a major life-threatening complication (e.g., intracranial bleeding), early palliative care referral and withdrawal of support should be considered.
According to the 2018 international summary report of the ELSO, based on > 15,000 ECMO runs for cardiac failure, overall survival is 55%. This survival varies considerably based on the indication for support. A recent metaanalysis by Cheng and colleagues of published cohort studies, including those that studied mixed causes of cardiogenic shock, showed that survival to discharge varies from 20% to 65%, with 5%–52% of the patients bridged from ECMO to a VAD and 4%–21% bridged to heart transplant, depending on the series ( Table 6.2 ). Among patients with cardiogenic shock secondary to an AMI, survival has increased to approximately 59% in specialized centers, and the strongest predictors of mortality are age older than 65 years, body mass index greater than 32 kg/m 2 , and international normalized ratio (in the absence of warfarin) of greater than 2 at the time of cannulation. The benefits of ECMO use in patients with AMI are likely due to an early stabilization of the patient, allowing early revascularization. In an observational study of patients with ST-segment elevation myocardial infarction and cardiogenic shock who underwent percutaneous coronary intervention (PCI) with or without ECMO support, those receiving ECMO had significantly lower 30-day mortality (39.1% vs. 72%; P = 0.008). The interpretation of these data is limited, however, by an era effect and possible confounding variability in coronary stent use among the groups. In a recent analysis by Esper and colleagues of patients in the catheterization lab supported with ECMO for acute coronary syndrome complicated by cardiogenic shock, 83% underwent successful percutaneous revascularization, with a 67% survival to discharge and 55% survival at 6 months. Interestingly, close to 20% of these patients required support with a durable VAD during their original hospitalization.
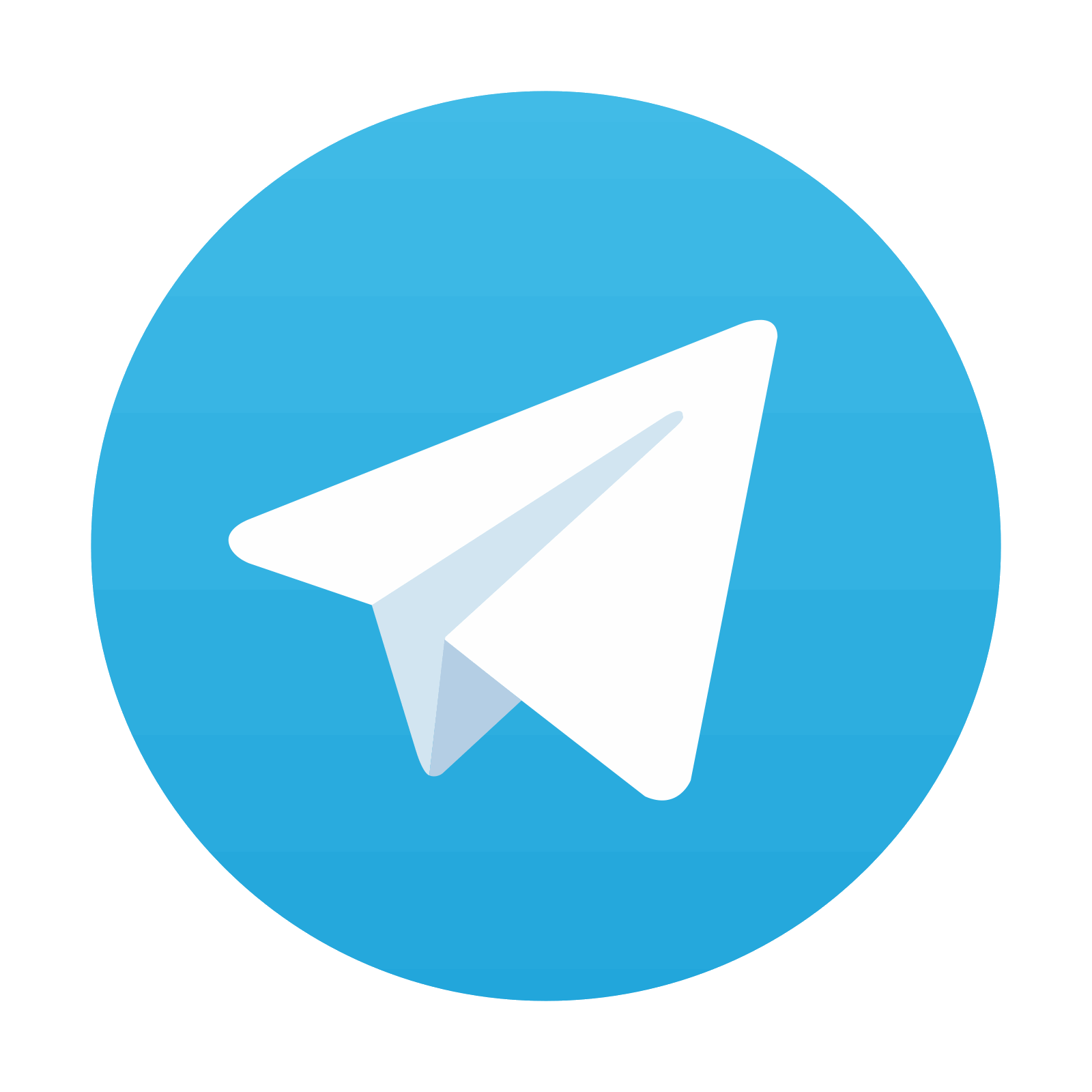
Stay updated, free articles. Join our Telegram channel
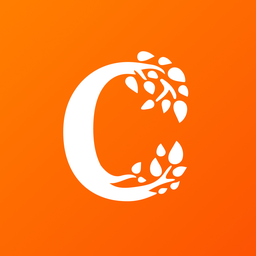
Full access? Get Clinical Tree
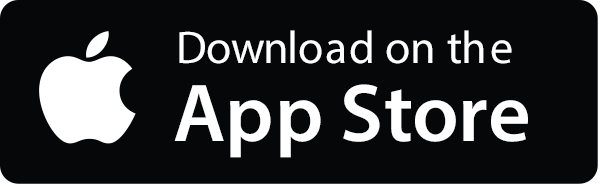
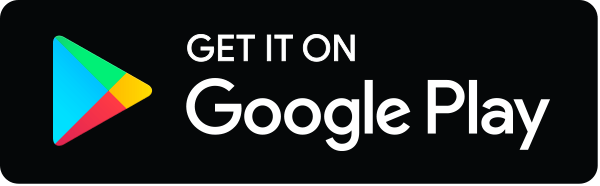
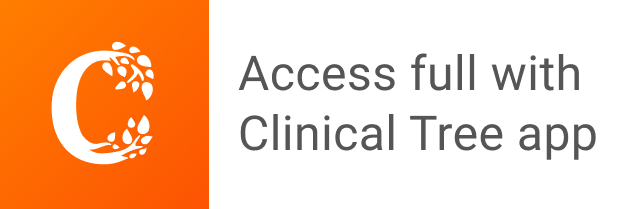