Fig. 1.1
A reproduction of Galen’s scheme. In Galen’s schema, the venous, arterial, and nervous systems, with the liver, heart, and brain as their respective centers, were separate, and each distributed through the body one of the three pneumata: respectively, the natural, vital, and animal spirits. Blood was carried both within the venous system and the arterial system. The heart and lungs worked together, with some of the blood passing through the pulmonary artery into the lungs; there it nourished the lungs and also mixed with the air breathed in. Some of the blood in the heart passed from right to left through “pores” in the interventricular septum. It was bright red because it had the vital spirit infused within it; from the left heart, it went out via the aorta to warm up the body
For at least the next 1,000 years, there were no apparent major discoveries involving the pulmonary circulation. Michael Servetus, the Spanish physician and theologist, was credited with the first description of the pulmonary circulation in his book “Christianismi Restitutio,” published in 1553 [5]. “The vital spirit has its origin in the left ventricle of the heart, the lungs especially helping towards its perfection. It is generated through the commingling which is effected in the lungs of the inspired air with the elaborated subtle blood communicated from the right ventricle to the left [5].” Servetus trained in Paris with Vesalius and was considered an exceptionally skilled dissector [4–7]. Sadly, he was regarded as a heretic and was ultimately tried in Geneva and burned at the stake [8]. His contributions were substantial. Only three surviving copies of the original document are known, although it was translated into a number of languages.
In 1924, an Egyptian physician, Muhyo Al-Deen Altawi, studying the history of medicine discovered a treatise by a physician, Ibn al-Nafis, entitled “Commentary on the Anatomy of Canon of Avicenna” in the Prussian State Library in Berlin [9]. These writings covered human anatomy, physiology, and pathology [9]. They were translated by two Syrian physicians, and it was learned that al-Nafis had made essentially the same observations as Servetus, in the thirteenth century, prior to several hundred years [9–11]. This work of Ibn al-Nafis was believed to be the earliest description of the pulmonary circulation.
Al-Nafis, a Syrian physician, trained in Damascus and ultimately practiced and researched in Egypt in the thirteenth century. He became the Sultan’s personal physician. Al-Nafis was credited as the first person to challenge the long-held belief of Galen that blood could pass through the cardiac interventricular septum; he was emphatic that all the blood that reached the left ventricle passed through the lungs [9–11]. He also stated that there must be small communications or pores between the pulmonary artery and vein (this concept preceded Marcello Malpighi’s discovery of the pulmonary capillaries by four centuries). Al-Nafis also postulated that nutrients for the heart are extracted from the coronary arteries [9–11]. Thus, Ibn al-Nafis and another prominent physiologist of the period, Avicenna (approximately 1000 A.D.), were among very few physician researchers to link the Galenic period in the second century to the European scientific Renaissance in the sixteenth century. The Haddad translation published in the Annals of Surgery in 1936 offers detailed descriptions of Al-Nafis’ discoveries [9]. In summary, Ibn al-Nafis should be regarded as a major influence and as the primary forerunner of Servetus, Vesalius, Colombo, and Harvey in the description of the pulmonary circulation as we now know it.
In the sixteenth century, in his book, “De Fabrica,” Vesalius described the pulmonary circulation in a way that very much resembled the description of Ibn al-Nafis [12]. In the first edition of his book in 1543, Vesalius agreed with Galen that the blood “… soaks plentifully through the septum from the right ventricle into the left …” [12, 13]. When he published his second edition in 1555, he omitted the above statement and wrote instead “… I still do not see how even the smallest quantity of blood can be transfused through the substance of the septum from the right ventricle to the left …” [12, 13]. Colombo, who studied under Vesalius at Padua, ultimately queried this concept, and determined through vivisection and dissection of human cadavers that “almost everyone assumes that the blood passes from the right ventricle to the left ventricle across this wall … But they are completely wrong. For the blood is conducted to the lungs by the pulmonary artery, where it is diluted and together with air is led to the left ventricle by the pulmonary veins, which no one has noticed until now, nor described in writing …” [12].
The work by Ibn al-Nafis, Servetus, and subsequently Vesalius and Colombo, paved the way for Harvey’s elegant description of the pulmonary circulation. “Exercitatio Anatomica de Motu Cordis et Sanguinis in Animalibus” (An Anatomical Exercise on the Motion of the Heart and Blood in Living Beings) is the best known work of William Harvey [14–17]. The book was first published in Latin in Frankfurt in 1628 and remains one of the most important works establishing concepts of the circulation of the blood. Harvey’s methods combined observations and experimental methods; among them were the examination of the effect of ligatures on blood flow. The book taught that blood was pumped around the body in a “double circulation,” so that after being returned to the heart, it was recirculated in a closed system to the lungs and back to the heart, where it was returned to the main circulation [14–17]. There was, however, one portion of the circulation which Harvey could not characterize, although he did postulate it, i.e., the way in which the veins and arteries subdivide into a capillary network. As alluded to above, it was in 1660, 3 years after Harvey’s death, that Marcello Malpighi of Bologna saw the blood moving in the capillaries of the frog’s lung, and thus the missing link that Harvey needed to fully characterize the circulation of the blood [18]. The period of the Islamic Golden Age, the long influence of the teachings of the Galen School, and the European Renaissance are shown on a timeline in Fig. 1.2.
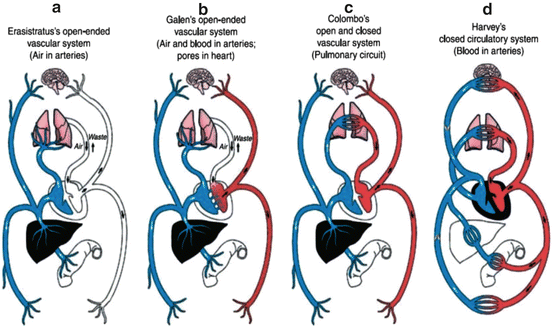
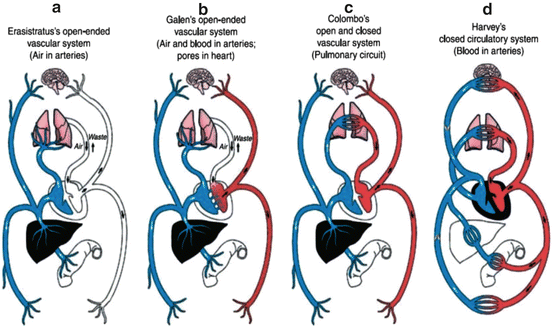
Fig. 1.2
Cardiovascular models over the course of time. (a) Erasistratus’ model, (b) Galen’s model, (c) Colombo’s model, (d) Harvey’s model. Reference: Arid WC. Discovery of the cardiovascular system: from Galen to William Harvey
Subsequent relevant discoveries included the demonstration by Boyle with his air-pump experiments in 1666 that air was essential to life [7]. The next year, Hooke demonstrated that it was the supply of air to the blood that was crucial to respiration [19]. In 1669, Lower found that the difference between arterial and venous blood was the change in color and that this change took place in the lungs and not the heart. In 1668, Mayow discovered that only part of the air, the “spiritus nitro-aereus,” was necessary for life [19]. Priestley, in 1774, called oxygen “dephlogisticated air.” In 1777, Lavoisier further clarified these concepts, demonstrating for the first time that during respiration, the air that was breathed in lost oxygen and gained “fixed air of Black.” (Black had discovered carbon dioxide 20 years prior.) Spallanzini subsequently characterized concepts involving tissue respiration [19].
Steven Hales deduced and published that the thinner walls of the right ventricle indicated that it had less work to do to force blood through the pulmonary circulation than the left ventricle did to maintain the systemic circulation [20]. In the early nineteenth century, Hope also studied the physics of the failing heart and described the symptoms and signs of heart failure, differentiating the effects of failure of the right ventricle from those of the left. By 1847, the work of Poiseuille together with Ludwig enabled blood pressure measurement [21].
Over the ensuing decades, pioneers in cardiopulmonary disease improved the means through which to access the pulmonary circulation and further characterize it. Werner Forssmann performed right-heart catheterization on himself in 1929, and thus demonstrated its feasibility in humans [22]. The catheter, however, was only advanced into the right atrium. Andre Cournand and Dickinson Richards developed catheters that could be advanced into the pulmonary arteries for pathophysiological studies of various congenital and acquired cardiac disorders [23, 24]. Drs. Forssmann, Cournand, and Richards received the Nobel Prize in Medicine in 1956 for their work [24]. Subsequent investigations advanced the field of right-heart catheterization and in 1970, balloon flotation flow-directed catheters that could be used at the bedside, without fluoroscopy, were introduced by Drs. Swan and Ganz [25–28]. These balloon flotation catheters were developed further for measuring all right-sided pressures, cardiac output (by thermodilution), and pulmonary capillary wedge pressure (PCWP), and for right atrial and right ventricular pacing [29, 30]. This technique evolved into, and still remains, the standard of care for diagnosing PH and characterizing its severity (Fig. 1.3).
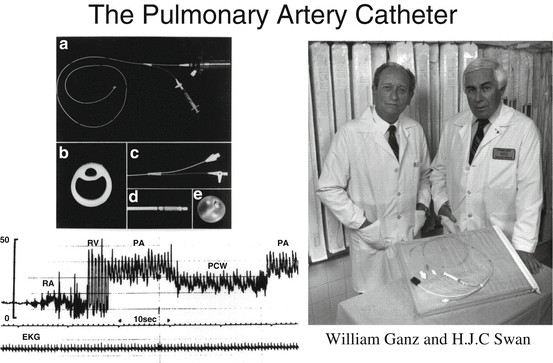
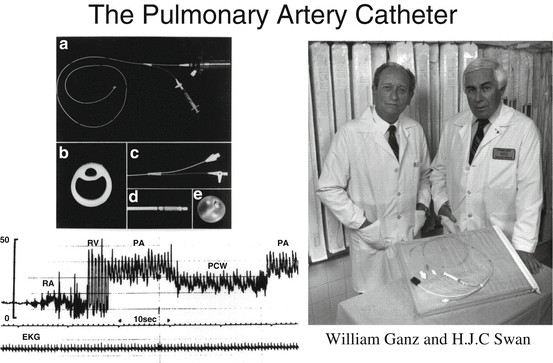
Fig. 1.3
In 1970, balloon flotation flow-directed catheters that could be used for right-heart catheterization at the bedside without fluoroscopy were introduced by Drs. Swan and Ganz (reproduced with permission from the American Heart Association)
The world’s first scientific conference dedicated to the pulmonary circulation was organized by the Chicago Heart Association and held in Chicago in 1958. This progress has been outlined in detail in a treatise arising from the Chicago conference [31].
Interest in the pulmonary circulation was furthered by the PAH epidemic in Europe caused by the diet drug aminorex [32]. As interest in PH evolved, international conferences organized by the World Health Organization (WHO) have taken place during which experts in the field have gathered to review the science and available literature as well as clinical practice experience in PH. Twenty-five years after the first meeting in Geneva, Switzerland, in 1973, a second WHO meeting on PH took place in Evian, France, in 1998 [33, 34]. Since that time, these international consensus conferences have taken place every 5 years, with the most recent meeting in Nice, France, in 2013. At these meetings, the classification, risk factors, and diagnostic and therapeutic approaches to PH have been progressively scrutinized and updated based upon international research efforts. Consensus statements/guidelines have been published based upon this work [35–37]. Modern-day PAH is rich with discoveries in both basic science and clinic research that have led to the development of at least four new drug classes of pulmonary vasodilators over the last 25 years that are now available to treat patients with PAH and other pulmonary vascular disease.
Structure and Function of the Pulmonary Circulation
The genes, molecules, cells, and tissues of the pulmonary circulation work in synchrony to determine function, with the primary function of the pulmonary circulation being to optimize the exposure of blood to alveolar air while maintaining a low enough resistance to accommodate passage of the full cardiac output. The lungs receive the entire cardiac output, with the normal cardiac index being approximately 3.5 L/min/m2. The lungs contain approximately 300 billion capillaries which supply approximately 300 million alveoli. The surface area of capillaries interfacing with alveoli for gas exchange is between 50 and 100 m2; this contact occurs at the 1–2 μm thick alveolar capillary membrane [38–40].
Importantly, at rest, in the absence of pulmonary vascular disease, the thin-walled right ventricle functions at a very low energy level; that is, the right ventricular (RV) afterload is such that this chamber is often described as merely a conduit. When an individual exercises, oxygen uptake (VO2) and carbon dioxide output (VCO2) increase by as much as 20-fold above resting values, and this increased gas exchange is matched by a substantial increase in cardiac output as emphasized above. In normal exercising individuals without left or right ventricular failure, the entire cardiac output is handled by the pulmonary circulation with neither pulmonary edema nor RV failure. Disease of structural components of the lung affecting blood flow through the pulmonary vessels at any level can lead to PH, and in turn result in RV dysfunction and failure. While both the pulmonary and the systemic circulations transport the same amount of blood, the pulmonary circulation is characterized by high flow and low pressure, with the average pulmonary vascular resistance (PVR) in the range of 1 mmHg × min/L (1 Wood unit) in healthy young adults. With normal aging, the PVR eventually increases to approximately 2–3 Wood units, and to 5–10 times higher with significant PH.
The calculation of total PVR is based upon Ohm’s law, and is calculated as the difference between mean pulmonary arterial (PA) and mean left atrial (LA) pressure, divided by the cardiac output (where LA pressure is approximated by PCWP); that is,
where mPAP = mean pulmonary artery pressure, mLAP = mean left atrial pressure, and CO = cardiac output.
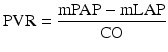
The diameters of small pulmonary arteries and capillaries account for the greater part of pressure drop across the pulmonary vascular bed. With exercise, significant elevation of PA pressure is prevented by recruitment of unopened capillaries and, to a smaller extent, distension of the elastic vessels [41]. Thus, at rest, a substantial portion of the capillary bed is unrecruited; some or all of these capillaries open when cardiac output increases in order to increase the total cross-sectional area of capillary bed, and decrease the PVR [42]. Because of this, when a healthy person exercises and the cardiac output is enhanced sixfold, the PA pressure increases only moderately [43]. The normally low pressure in the lung circulation prevents fluid from moving out from the vasculature into the interstitial/alveolar space. In pathologic states, when left-sided pressures are significantly elevated, this transudation can occur. With progressive elevation of the PA pressure in PAH, there is elevation of the RV pressure and for a period, the cardiac output is maintained. When the pressure rises too high, particularly over a relatively short period of time, compensation becomes impossible and RV failure ensures.
Modeling analyses have demonstrated the interface between vascular geometry and elasticity to pressure-flow relationships and PVR [44, 45]. Recruitment and distension mechanisms become markedly abnormal in PAH. Even early in PAH, when resting PA pressure is normal, these mechanisms impact upon exercise tolerance, and over time the inability to compensate even at rest results in marked dyspnea, that is, functional class IV symptoms. In PAH, the elevated pressure results from progressive vascular remodeling, vasoconstriction, and thrombosis of small pulmonary arteries and thus from the resistance increase.
Clinicians generally define RV afterload in terms of PVR and this measure has been used as a primary or secondary end-point in clinical trials. However, PVR reflects only the non-pulsatile component of blood flow, and neglects the significant contribution of compliance [44, 45]. Compliance takes into account the pulsatile components of the arterial load. Thus, compliance (or stiffness; the reciprocal of compliance) is an important factor contributing to systolic and diastolic pressure in the pulmonary circulation. In turn, systolic pressure determines systolic wall stress which represents the true ventricular afterload. In the early stages of PAH, a small increase in PVR will be accompanied by a relatively large drop in compliance. Later as the vascular disease progresses, the increase in PVR will continue but the decrease in compliance will be limited as vessel wall stiffness reaches a maximum.
The PVR can also be characterized by Poiseuille’s equation. Here, for laminar flow, the relationship of the resistance of a tube to the tube’s physical characteristics and viscosity of the perfusing fluid is described:
where R = resistance, L = tube length, r = tube radius, and η = viscosity of perfusion fluid.

Thus, based upon this equation, the crucial determinant of PVR is the vessel’s radius, since resistance is proportional to L/r 4. In the body, however, flow does not conform precisely to this relationship because it assumes long, straight blood vessels, a Newtonian fluid (e.g., water; blood is non-Newtonian), and steady, laminar flow. Nonetheless, the relationship clarifies the dominant influence of vessel radius on resistance and flow and thus relates how physiological vascular tone and pathological vascular stenosis dramatically affect pressure and flow.
To better conceptualize the hemodynamics of the pulmonary circulation in PAH, studies have investigated the measurement of PVR and compliance in healthy subjects and PH patients [46, 47]. Resistance and compliance in the pulmonary circulation have proven inversely related by a “hyperbola” [46]. It appears that PVR and compliance together describe RV afterload better than either of them alone. These concepts are discussed in more depth in Chaps. 3 and 5.
Biophysically based computational models offer the potential for integrating and analyzing experimental observations of the pulmonary circulation. These tools have evolved to sophisticated models that predict integrated structure-function relationships. In order to facilitate a more detailed analysis of structure-function relationships in the pulmonary circulation Burrowes and colleagues developed an anatomically based finite element model of the pulmonary vasculature. This model represents the complex geometry found in the pulmonary circulatory system for application in future, more detailed structure-functional investigations [45]. Multidetector row computed tomography (MDCT) imaging was utilized together with a volume-filling branching algorithm and an empirically based algorithm to generate the supernumerary vessel geometry. Analysis of branching properties and geometric parameters demonstrated close correlation between the model geometry and anatomical measures of human pulmonary arteries and veins. A review of mathematical and computational models of the pulmonary circulation has recently been published [48].
The Distribution of Blood Flow in the Lung
In the 1960s, experimental studies in isolated lung models indicated that blood flow distribution in the zonal model was gravitationally dependent, with lung tissue in the nondependent apical upright lung receiving proportionately less cardiac output than the dependent lung bases [49]. Perfusion is defined by the arterial-venous gradient. In zone 1, where mPAP is essentially zero, the alveolar pressure is greater than either the arterial and venous pressures and so there is collapse of the blood vessels, preventing perfusion. In zone 2, the mPAP is greater than alveolar pressure and perfusion is defined by the arterial-alveolar gradient. In zone 3, both mPAP and pulmonary venous pressure are greater than alveolar pressure. This zonal concept had been the accepted model for decades. More recent studies however have emphasized irreversibility of the flow gradient with reversal of posture [50] and “isogravitational heterogeneity of flow” [51]. These results do not suggest a theory of flow distribution that is purely gravitational. These findings together with other investigations imply that the hydrostatic effects of gravity are not the only factors determining the distribution of pulmonary blood flow [50–52]. Furthermore, imaging studies have suggested that gravitational effects on the regional distribution of pulmonary blood flow occur primarily via deformation of parenchymal tissue rather than by the balance of microcirculatory pressures, as dictated by the classic zonal model [50–52]. The potential contribution of each of these mechanisms remains unclear [53, 54], but modern studies in large animals suggest that the effect of gravity on regional blood flow in the lung is relatively low and that the primary determinant of flow is vascular resistance.
Hemodynamic Comparisons with the Systemic Circulation
In contrast to the distribution of compliance in the pulmonary circulation, the number of arterioles in the systemic circulation is approximately ten times less, with resistance ten times higher and compliance ten times lower. Thus, in the systemic circulation resistance is mainly located in the distal small arteries and arterioles, with compliance mainly located in the aorta [46, 55]. In the pulmonary circulation, arterial compliance is distributed over the entire arterial tree. This means that occlusion of a whole lung or lobe or segment not only increases resistance but also decreases compliance.
The ranges of pressures in systemic hypertension are relatively small compared to the very large range of pressures in PH. As patients age with systemic hypertension, resistance increases by only about 20 % whereas compliance can decrease by threefold. In PH, however, resistance can increase and compliance can decrease by a factor of 20.
The concept of oscillatory power fraction (ratio of oscillatory to mean power) has been used to characterize reactive circulatory beds. The oscillatory power fraction of the left ventricle is only about 10–15 % and increases in the setting of systemic hypertension [46, 56]. Oscillations play a more important role, however, in the pulmonary vascular bed so that the RV oscillatory power fraction is twice that of the systemic vascular bed. However, in contrast to systemic hypertension oscillatory power fraction remains constant in PH [57]. A more exhaustive discussion of components of the arterial load on the RV in terms of resistance and arterial compliance, and the consequences of load changes for RV work and function, has been published [46].
Thus, the pulmonary circulation is a low-resistance, highly compliant vascular bed that is markedly influenced by pressures generated by the heart and the thorax. Based on the intrinsic properties of the pulmonary circulation and its relatively insignificant potential for vasomotor control, relatively modest external forces can exert relatively large hemodynamic effects, making attempts to distinguish between active and passive changes in vessel size difficult. Detection and characterization of such vasomotor activity require substantial attention to the effects of respiration and cardiac activity on vascular pressures [58, 59].
Neural and Humoral and Regulation of the Pulmonary Vasculature
Adrenergic and cholinergic efferent nerves innervate the pulmonary circulation. The concentration of nerve fibers is greatest in larger vessels and at vascular bifurcations and is less influential in smaller vessels. Stimulation of α-adrenergic receptors mediates vasoconstriction, while β-adrenergic receptors mediate vasodilation [60]. α-Adrenergic mechanisms appear to contribute little to normal pulmonary vasomotor tone; blockade of these receptors modifies neither baseline pulmonary vasomotor tone nor the hypoxic response. Because pulmonary vessels are normally in a dilated state, β-adrenergic responses are not evident. However, β-adrenergic blockade does enhance the vasoconstrictor response to catecholamines, which stimulate both α- and β-receptors, and increases in vascular tone enhance responses to β-adrenergic agents [60]. In summary, the pulmonary vascular bed is quite richly innervated and yet stimulation of these nerves produces relatively small changes in vasomotor tone. Perhaps the influence of vasodilators such as nitric oxide (NO) and prostacyclin normally predominate, minimizing the vasoconstrictor effect of the sympathetic nerve stimulation [61].
A number of mediators have the potential to affect the pulmonary circulation [62]. Vasoconstrictors include endothelin, serotonin, histamine, thromboxane, leukotrienes C4 and D4, angiotensin II, norepinephrine, and platelet-activating factor. These mediators bind to specific receptors on pulmonary vascular smooth muscle cells and induce smooth muscle contraction via activation of second messenger pathways. Key pulmonary vasodilators include NO, prostacyclin, acetylcholine, and bradykinin [62]. NO plays a key role in regulating the changes in pulmonary vasomotor tone induced by these vasoconstrictors [61]. The NO, endothelin, and prostanoid pathways have been by far the most influential mediators in the evolution of PAH therapy.
PAH is associated with impaired production of NO [63]. NO is produced by many cell types in the lung and plays an important physiologic role in the regulation of pulmonary vasomotor tone by several known mechanisms. NO stimulates soluble guanylyl cyclase, resulting in increased levels of cyclic GMP in pulmonary vascular smooth muscle cells leading to increased activation of cGMP-dependent protein kinase resulting in pulmonary vasodilation [64, 65]. In addition to regulating vascular tone, NO and cGMP signaling can also inhibit proliferation and induce apoptosis in vascular smooth muscle cells [66, 67]. Based upon the NO pathway, drug therapy in PAH continues to be explored with phosphodiesterase-5 inhibitors such as sildenafil and tadalafil that inhibit the metabolism of cGMP and soluble guanine cyclase stimulators such as riociguat that increase cGMP synthesis, and more recently, with nitric oxide itself [68–70].
The endothelin system has been extensively studied since its discovery by Yanagisawa and colleagues in 1988. Endothelin-1 (ET-1) is a powerful vasoconstrictor and proliferative cytokine, serving as a key mediator in pulmonary vascular biology, and an important mediator of PAH. Plasma ET-1 levels are elevated in patients with PH [71]. Expression of ET-1 mRNA and protein is increased in the endothelial cells found in vascular lesions in idiopathic PAH [72]. ETA receptors are found in smooth muscle cells and ETB receptors are located in both endothelial cells and smooth muscle cells. ET-1 is released from endothelial cells and acts primarily on underlying smooth muscle cells. Several ET-driven processes appear involved in lung vascular and structural remodeling. These include smooth muscle vasoconstriction and proliferation, an effect on fibroblasts resulting in contraction, proliferation and fibrosis, and finally an effect upon the endothelium itself causing proliferation, vasodilation (mediated through NO and prostacyclin), and vasoconstriction (via thromboxane A2) [73]. Evidence suggests a role for autocrine/paracrine signaling in the ET system and PAH pathway [74]. ET receptor antagonists have clearly proven beneficial in PAH [68].
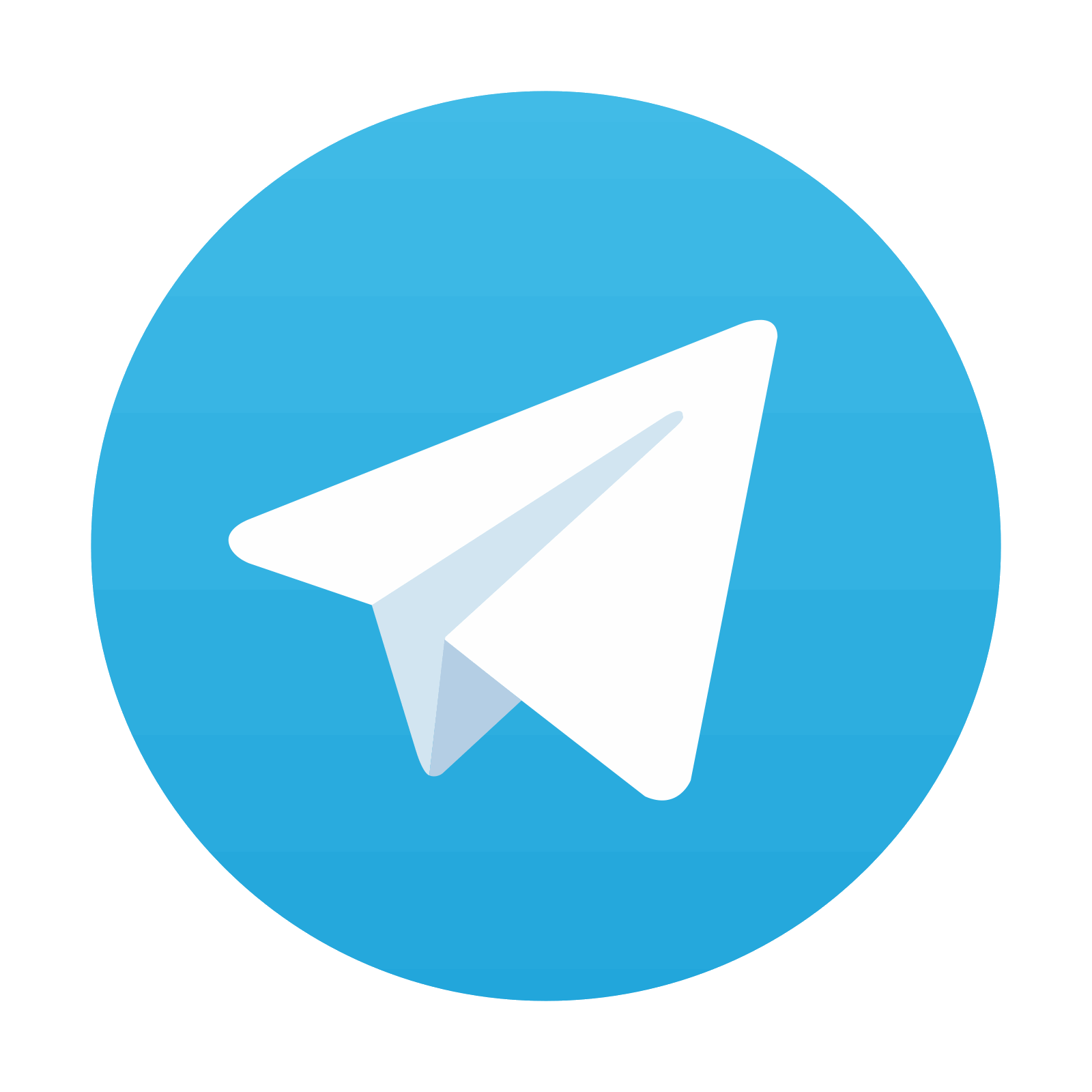
Stay updated, free articles. Join our Telegram channel
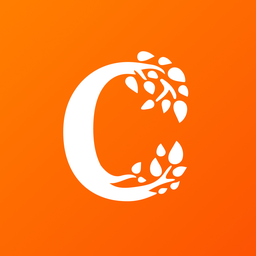
Full access? Get Clinical Tree
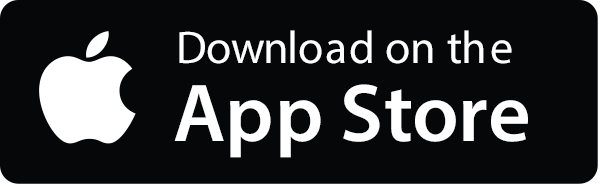
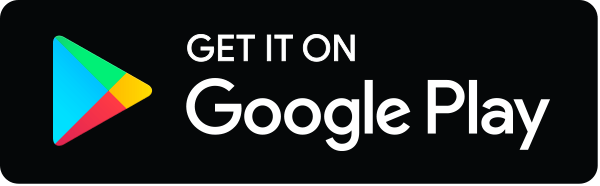