, Dilip R. Karnad2 and Snehal Kothari3
(1)
Cardiac Safety Services Quintiles, Durham, North Carolina, USA
(2)
Research Team, Cardiac Safety Services Quintiles, Mumbai, India
(3)
Cardiac Safety Services Global Head, Cardiac Safety Center of Excellence Quintiles, Mumbai, India
One of the most feared complications in medicine is sudden death caused by drug-induced proarrhythmia. Accordingly, concerted efforts have been made to define a drug’s proarrhythmic potential before regulatory approval (Link et al. 2010).
7.1 Introduction
The first step in the field of proarrhythmic cardiac safety is to determine to the greatest degree possible during nonclinical and preapproval clinical development whether a noncardiac drug has the propensity to lead to the polymorphic ventricular dysrhythmia torsades (Dessertenne 1966) in patients who may be prescribed the drug should it subsequently be approved for marketing. The second step is to remain alert to unexpected cardiac adverse drug reactions during its therapeutic use. This chapter’s focus is the first step.
Drug-induced arrhythmogenesis can result in nonfatal dysrhythmias causing syncope, and on rare occasions it can prove fatal. Proarrhythmic cardiac safety concerns led to the marketing withdrawal of various drugs in the United Kingdom (UK) and the United States (USA) from the late 1980s to the early 2000s. These included terodiline (indicated for urinary incontinence, withdrawn from both markets in 1991), the antihistamine terfenadine (withdrawn from the US market in 1998), and levacetylmethadol (indicated for opiate addiction, withdrawn from the UK market in 2003) (Turner et al. 2013).
As first noted in Sect. 1.4.1, regulatory concern with these marketing withdrawals led to the release in 2005 of two ICH guidelines: ICH S7B, which focuses on nonclinical assessments of relevance to the proarrhythmic liability of noncardiac drugs (ICH 2005a), and ICH E14, which addresses preapproval clinical assessments and introduced the thorough QT/QTc (TQT) study that was discussed briefly in Sect. 5.5.1 when summarizing the relevant statistical considerations and techniques (ICH 2005b). ICH S7B is introduced in this chapter, and ICH E14 is discussed in greater detail.
The first part of this chapter’s title, “The Proarrhythmic Cardiac Safety Regulatory Landscape circa 2005–2015,” provides a clue that, as we write this chapter in January 2016, this landscape is in a state of transformation. As discussed in the following two chapters, initiatives are underway to modify both nonclinical and preapproval clinical assessments of a drug’s proarrhythmic liability. Since an alternative approach in the preapproval clinical domain is closer to formal regulatory approval, this is discussed in Chap. 8: an alternative nonclinical approach is then discussed in Chap. 9. Nonetheless, the topic of this chapter, cardiac safety circa 2005–2015, remains of considerable importance. Events and regulatory science discussed herein address the origins of the formalized domain of proarrhythmic cardiac safety, a decade’s work in this domain, and reasons why modifications to the ICH S7B and ICH E14 regulatory landscape have been deemed desirable.
7.2 A Brief History of Proarrhythmic Cardiac Safety
Table 7.1 presents a timeline of important events in the development of the current landscape, and, since methodologies and regulatory landscapes are evolving as we are writing this chapter, the potential future proarrhythmic cardiac safety regulatory landscape.
Table 7.1
Timeline of important events in the evolution and employment of the thorough QT/QTc study as described in ICH Guideline E14
Date | Event |
---|---|
1997 | The European Medicines Evaluation Agency’s (EMEA’s) Committee of Proprietary Medicinal Products released a “Points to Consider” document on the assessment of the potential for QT interval prolongation by noncardiovascular medicinal products (EMEA 1997) |
1999 | The FDA set up a working group and generated internal documents on QT assessment |
2001 | Health Canada submitted a concept paper to the FDA |
2003 | A joint Health Canada/FDA Concept Paper was circulated and the ICH process initiated |
2005 | ICH issued guidelines S7B and E14, which were adopted in Europe and the USA in 2005 and in Canada in 2006 |
2006 | FDA established its QT interdisciplinary review team (IRT), which reviews protocols and study reports for thorough QT/QTc studies and advises review divisions accordingly |
2006 | Health Canada released regional guidance documents to support the interpretation and implementation of ICH E14 |
2008/2012/2014 | The ICH E14 working group released a “questions and answers” document in 2008, which was expanded with additional questions and answers in a revised document issued in April 2012 and revised again in March 2014 |
2009/2010 | The Japanese Pharmaceuticals and Medical Devices Agency (PMDA) adopted ICH E14 in 2009, allowing a year’s “grace period” before its full implementation in November 2010 |
2015 | The ICH E14 working group released the third revision of the “questions and answers” document associated with ICH Guideline E14 in December 2015 (ICH, 2015). This revision is triggering a major change in the cardiac safety regulatory landscape |
The development of the regulatory science employed in this field can be said to date back to the release in December 1997 of a “Points to Consider” document by the European Medicines Evaluation Agency (EMEA, now known as the EMA) which addressed the assessment of the potential for QT interval prolongation by noncardiovascular medicinal products (EMEA 1997). The field of proarrhythmic cardiac safety was formalized by regulatory agencies’ adoption of ICH S7B and ICH E14. ICH E14 introduced the TQT study. A “Questions and Answers” document prepared by the ICH E14 Working Group containing questions and associated answers was released in June 2008, a revised version containing additional questions and associated answers was released in April 2012, and an additional revision released in March 2014. The third revision, referred to here as ICH E14 Q&A R3, was released in December 2015 (ICH E14 Q&A R3, 2015).
Across the last two decades or so, cardiac safety has been a major concern in the development, approval, and marketing of new drugs (Turner et al. 2013; Kothari et al. 2015), with a substantial number of drugs being restricted in their clinical application or withdrawn from the market due to adverse cardiovascular effects. There were 47 instances of postmarketing withdrawal of drugs between 1957 and 2007; 45 % of these were due to concerns regarding cardiovascular toxicity (Redfern et al. 2010). Similarly, 27 % of the potential new drug molecules that failed in nonclinical investigations in the last two decades did so because of cardiovascular toxicity (Turner et al. 2014). Consequently, significant attention has been focused on the prospective exclusion of unacceptable cardiovascular risk during drug development. The level of risk that is deemed acceptable differs based on the disease for which a drug is being developed, the relative severity of the adverse cardiovascular effects, and the availability of safer therapeutically beneficial alternatives.
Among the possible cardiovascular risk liabilities, the risk of drug-induced torsades has been a major reason for the withdrawal of licensed drugs, accounting for around 26 % of drugs withdrawn from the market between 1990 and 2005 (Valentin 2010). This risk was not identified prospectively during the development of these drugs given the relative rarity of these events and the limited number of clinical trial participants studied in the preapproval period. However, a common thread which subsequently emerged in these cases was their association with prolongation of the QT interval component of the ECG. A stylized representation of the ECG, the QT interval, and QT interval prolongation was presented earlier as Fig. 3.2.
It was also apparent that these occurrences were concentration related and almost exclusively linked to delayed cardiac repolarization due to drug-induced inhibition of the rapid delayed-rectifier potassium current (I Kr), the main repolarizing current in ventricular cardiomyocytes (Hancox et al. 2008; Salvi et al. 2010). As discussed in Chap. 2, this current occurs due to an efflux of potassium ions through the I Kr channel encoded by hERG, which is therefore also referred to as the hERG channel (Heijman et al. 2014). The idea therefore arose that the proarrhythmic liability of drugs could be prospectively investigated during drug development by using the degree (if any) of QT interval prolongation as a surrogate for their propensity to delay cardiac repolarization. Thus, the preapproval proarrhythmic cardiac safety testing paradigm described in this chapter came to be primarily based on the predictive link between drug-induced hERG channel blockade observed during in vitro nonclinical studies and QT interval prolongation in human participants in clinical trials.
7.3 Nonclinical Proarrhythmic Cardiac Safety Investigations: ICH S7B
The question of interest in this domain can be expressed as follows: can a nonclinical test, or more likely a battery of tests, correctly identify drugs that will, and will not, have a proarrhythmic liability in humans? Failing to identify a drug compound that is proarrhythmic in humans is far from desirable, but so is “identifying” a compound that would not have been proarrhythmic in humans, and thereby terminating a compound that potentially could have been an efficacious and acceptably safe drug. This point is discussed in more detail in due course.
Since its release in 2005, ICH S7B has governed the nonclinical proarrhythmic cardiac safety regulatory landscape. Two central components of this landscape are the in vitro hERG current assay and the in vivo QT prolongation assay. Although delay of repolarization can occur through modulation of several types of ion channels, inhibition of hERG current is the most common mechanism responsible for drug-induced QT interval prolongation and hence the focus of ICH S7B. The guidance listed the following objectives:
Identify the potential of a drug molecule (and its metabolites) to delay ventricular repolarization.
Relate the extent of the delayed repolarization to the concentration of the drug molecule and its metabolites.
Elucidate the mechanism of action of the delayed repolarization.
In conjunction with other relevant information, estimate the extent of delayed repolarization and QT prolongation in humans.
In vitro and in vivo methodologies can obtain information at several functional levels:
Ionic currents measured in isolated animal or human cardiac myocytes (or cardiomyocytes), cultured cardiac cell lines, or heterologous expression systems for cloned human ion channels
Action potential parameters in isolated cardiac preparations or specific electrophysiological parameters indicative of action potential duration in anesthetized animals
Proarrhythmic effects measured in isolated cardiac preparations or animals
ECG parameters measured in anesthetized or conscious animals
In vitro electrophysiology studies can provide information about the drug’s effects on hERG current (and other cardiac ionic currents), thereby helping to identify cellular mechanisms of action affecting repolarization. Human cardiac ion channel proteins can be expressed in heterologous expression systems (noncardiac cells) to assess the drug’s effect on specific individual ion channels in isolation from others (Leishman and Waldron 2006). hERG channels are typically heterologously expressed in Chinese hamster ovary (CHO) cells or human embryonic kidney cells. Xenopus oocytes have been used, notably as a demonstration of the hERG blocking action of terfenadine, but drug access may be limited in this assay, making mammalian cell lines and CHO cells preferable (Brown 2005).
An important concept in this context is the drug’s half maximal inhibitory concentration (IC50). The IC50 indicates how much of the drug is needed to inhibit specific biological activity in vitro by half. Considerable care must be taken in the assessment of IC50 since, in the determination of the safety margin, which puts hERG liability in context, it is divided by the effective therapeutic plasma concentration (ETPC) of the unbound drug. hERG IC50/ETPC ratios greater than 50–100 are generally associated with drugs where the results from a TQT study are not of regulatory concern, and there is a lack of a QT liability during therapeutic use. While lower values of the ratio can be poorly predictive, the majority of drugs for which there are no reports of TdP in humans have ratios greater than 30 (Redfern et al. 2003).
7.3.1 An Example of a Battery of Nonclinical Tests
One example of a battery of nonclinical tests was proposed by Bass and colleagues in a 2008 publication discussing an initiative from the Health and Environmental Sciences Institute (HESI), a division of the International Life Sciences Institute (Bass et al. 2008). The authors noted that “the critical challenge in the pharmaceutical industry today is to identify experimental models, composite strategies, or biomarkers of cardiac risk that can distinguish a drug which prolongs cardiac ventricular polarization but is not proarrhythmic, from one that prolongs the QT interval and leads to TdP.” They observed that a problematic feature of the biomarkers of proarrhythmic risk discussed in both ICH S7B and ICH E14 is their low specificity and hence “the potential for promising new test agents to generate false-positive results with a high frequency,” an issue discussed in more detail in due course.
The paper by Bass and colleagues summarized the objectives of a workshop entitled “Moving towards better predictors of drug-induced Torsade de Pointes” that brought together experts in the field to work collaboratively towards a better fundamental understanding of the emerging science, trends, and methodologies relating to the prediction of drug-induced TdP. These objectives included the following (Bass et al. 2008):
Identify the mechanisms for drug-induced TdP to help develop better tools for identifying drugs carrying this liability.
Evaluate emerging nonclinical methodologies for predicting drug-induced TdP.
Identify biomarkers in nonclinical studies that may be applied to clinical testing for drug-induced arrhythmia.
Identify critical aspects of nonclinical and clinical methods of evaluating the potential for drug-induced TdP in the context of public health decision-making.
Identify short- and long-term priorities for developing better predictors of drug-induced TdP.
The authors concluded that there was a need for both in vitro and in vivo TdP proarrhythmia models to help increase knowledge of arrhythmogenic mechanisms and substantially improve the predictive value of a nonclinical battery of tests for the clinical proarrhythmic liability of new drugs. They proposed a multifaceted strategy employing test systems of increasing complexity (i.e., concurrently assessing several parameters at a time), including single cells, isolated cardiac tissue, isolated hearts, intact animals, and diseased animals.
7.4 Preapproval Clinical Investigations of Proarrhythmic Liability
Before discussing the TQT study in detail, it is appropriate to consider how ECGs are collected during the study and also how they are analyzed after the study has been completed. Both the acquisition of digital ECGs and their analysis require a combination of several logistical considerations, equipment-related procedures, and data management steps that the investigational site collecting the waveforms and the laboratory (lab) analyzing them must implement.
7.4.1 Collection of High-Fidelity Digital ECG Waveforms
Acquisition of high-fidelity digital ECGs is achieved in one of two ways: employment of a 12-lead resting ECG device that records 10-s strips of ECG signals or use of a 12-lead Holter device that records continuous ECG signals in ambulatory participants for 24 h or longer. At the time of drafting ICH E14, ambulatory ECG monitoring was not considered to be sufficiently well validated to be used as the primary methodology employed in the assessment of drug-induced effects on the QTc interval. Therefore, a significant number of early TQT studies used 12-lead resting ECG recordings. Given the advances in Holter ECG recording technology, it has been demonstrated that Holter ECGs can be as accurate as 12-lead digital ECGs in the assessment of drug-induced changes in QT and RR intervals. Consequently, use of 12-lead Holter recorders in TQT studies steadily increased. TQT studies therefore now often employ Holter devices for collection of ECG waveforms, since this approach enables continuous digital waveforms to be recorded and short sections of 12-lead ECG strips to be extracted at prespecified study time points. This approach incorporates the advantage of selecting ECGs that are relatively free of artifacts and that are attained at a stable heart rate. Moreover, these 24-h recordings have a 50–100 times higher likelihood of detecting transient cardiac arrhythmias than conventional 10-s ECGs (Min et al. 2010).
The digital ECG waveform can be considered as a series of dots, with the precision of measurement afforded corresponding to the sampling frequency of the ECGs. Digital ECG recorders with a sampling frequency of 500 Hz (samples that are 2 msec apart) to 1000 Hz (samples are 1 msec apart) are optimal. In early TQT studies employing 12-lead Holter ECGs, the sampling rate employed was considerably lower, around 180 Hz, because of technological limitations at that time. However, Holter recorders used more recently have sampling rates of 500 Hz and 1000 Hz, equal to those of 12-lead resting ECG devices (Panicker et al. 2010).
Consistency in operator techniques relating to skin preparation, ECG lead placement, and the study participants’ position are important components of the collection of good-quality ECGs, since inconsistencies in recording methodology add to variability in ECG parameter values. Site training to provide clear instructions that ensure participants rest in a supine position for several minutes prior to the ECG acquisition time points to ensure a stable resting heart rate is essential: otherwise, activity-related heart rate changes could be falsely attributed to the study drug. Additionally, ECG recording must precede the drawing of blood for pharmacokinetic (PK) samples for exposure–response analysis, a topic discussed in the following chapter.
In studies using 12-lead resting ECG devices, the digital ECG files recorded at the site are transmitted via an in-built modem to the ECG receiving system on the server of the lab where they will be analyzed. After the confirmation of demographics, ECG files are converted into FDA-compliant Extensible Markup Language (XML) files that are conveyed to the ECG analysis software at the lab. When employing 12-lead Holter devices, the digital ECG data are recorded on a removable memory card that may be sent via a courier to the lab. The digital Holter file is downloaded and used for the extraction of discrete 10-s ECG files that are analyzed by a process similar to that used for 12-lead resting ECG. Alternatively, the digital Holter recording from the removable memory card may be transferred to the investigational site computer and transmitted to the lab’s server via a secure web upload.
7.4.2 The Core ECG Lab
The labs that analyze ECGs collected during a TQT study are highly specialized for this purpose and are referred to as core ECG labs. This nomenclature is similar in intent to the term central lab: central labs are used to analyze different types of biological samples (e.g., blood and urine) taken during many large clinical trials. The name central lab refers to testing labs to which samples from all of the investigational sites in a clinical trial are shipped. This strategy has two important advantages compared with using a large collection of local labs, i.e., laboratories close to each investigational site:
Assurance that the lab conducting the analyses of the samples is compliant with current good clinical practice (cGCP) is much easier.
Statistical difficulties associated with analyzing pooled lab data are avoided.
cGCP guidances require that all labs have data-audit trails, standard procedures, trained staff, archives of samples and data, and routine quality assurance inspections (Prokscha 2007). If multiple labs were used, assurance would be required that cGCP requirements were met at every one. Use of a single central lab makes assurance of cGCP much simpler.
Analysis of data that have been collected from many sources and then pooled into one data set can lead to considerable statistical problems. Since the majority of lab measurements are surrogates and reference ranges can vary from analytical method to method, many different types of errors can occur during lab testing. These can be due to variation in many aspects of data collection, including the technician, an instrument, the environment, and the reagents used. While statistical approaches to standardize values from several local laboratories, each with their own reference ranges, have been described (Chuang-Stein 1992), central labs provide a real statistical advantage: all samples are analyzed in the same manner in the same laboratory, thereby providing a much greater degree of fidelity. Thus, the role of the ECG core lab is not restricted just to ECG analysis as discussed in the next section but also encompasses aspects of ECG acquisition and processing as well as submission of the annotated digital ECG data to regulatory agencies.
7.4.3 Analyzing ECG Waveforms in a Core ECG Lab
ICH E14 recognizes that the threshold of regulatory concern in a TQT study is small, i.e., a mean QT prolongation of about 5 msec. Given that the length of a “typical” QT interval is in the order of 400 msec, the increase of interest is in the order of 1 %. Detecting such a small change requires a high degree of accuracy and precision in ECG measurement. ICH E14 recommends skilled readers without specifying the specific training needed. A technician performing the initial read followed by a cardiologist overread is considered adequate. It is the responsibility of the core lab to have appropriate criteria for reader selection and training and benchmarking their competence (Panicker et al. 2009). ICH E14 further states that the ECGs must be analyzed by a small number of readers and that all ECGs from the same participant be read by the same reader (blinded to treatment arm) to maintain consistency in QT measurement. A specific assessment of reader variability is also required, with a subset of the ECGs being reread to quantify inter- and intra-reader variability. Each core lab should have a well-defined approach to quantifying reader variability (Salvi et al. 2014). Without appropriate care, a QT interval measured in the same ECG by the same reader blinded to his or her previous measurement may vary up to 25 msec (Malik and Camm 2001).
The method of QT interval measurement is also of great importance (Salvi et al. 2010). ICH E14 recognizes that there are several different methodologies for the measurement of ECG intervals which differ in “what to measure” in terms of items and characteristics including conventions for lead selection, waveform presentations, definition of T-wave offset, and handling of U-waves. It is not prescriptive about the method chosen but emphasizes the need to use a consistent approach. In this context, the use of a positive control such as moxifloxacin, a drug whose effects on ECG waveforms are very well known, is an internal validation of the QT interval measurement methodology and the accuracy of the ECG readers.
A core lab’s ECG analysis system also enables various techniques of measurement. ICH E14 recommends one of the following approaches for TQT studies:
Fully manual method. Identification of waveforms and placement of fiducials (the onset of the QRS complex and the offset of the T wave) are performed entirely by the human reader without any assistance from a computer.
Manual adjudication (semiautomated/computer assisted). Initial identification of waveforms and placement of fiducials are performed by a computer algorithm. This is followed by a 100 % overread by humans who can either accept or alter the placement of fiducials as deemed appropriate. This approach is most commonly used.
Regardless of which technique is used, readers should be trained in, and follow, standard operating procedures with prospectively defined criteria on how to place or correct fiducials for interval measurement. Although fully automated methods are available and offer the advantage of being consistent and reproducible, they have not yet been sufficiently validated and can yield misleading results, especially for less-than-good-quality ECGs and in the presence of morphologic or rhythm abnormalities. However, with improvements in computer algorithms, there are now validated computer-assisted techniques, often described as highly automated reading, wherein intervals are measured by a computer algorithm that also provides confidence scores based on the quality, morphology, and measured values. Only select ECGs exceeding prespecified quality cutoffs then undergo human overread. The ECG readers also manually assess the shape of the T wave for any drug-induced changes and for the presence of other diagnostic features such as cardiac arrhythmias and conduction abnormalities. A combination of validated technology solutions, trained readers, and scientifically rigorous methods is therefore essential to ensure high-quality ECG data.
7.4.4 Adjusting QT Measurements for Heart Rate
QTc values are used in analysis of potential drug-induced QT interval prolongation, where the “c” stands for corrected for heart rate. Many correction factors have been developed to address this issue (see Camm et al. 2004). The more commonly used correction factors, QTcB (Bazett’s correction) and QTcF (Fridericia’s correction) employed in this manner date back almost 100 years in the context of clinical practice.
When discussing the history of the reasons behind the choice of the preferred formula for adjusting QT interval values, Camm and colleagues commented as follows (Camm et al. 2004, p 44):
Of all the formulas used in the past, the most commonly used are Bazett’s square-root formula (QTc = QT/RR1/2) and Fridericia’s cube-root formula (QTc = QT/RR1/3). Between the two, Bazett’s formula is more commonly used [in clinical practice] and most reported values are given using Bazett’s formula because of its simplicity (most simple calculators have a function for a square root but not for a cube-root computation, which gives a practical advantage to Bazett’s over Fridericia’s correction).
Bazett’s correction is still the most commonly used correction factor in clinical practice: most ECG machines used in clinical practice settings automatically provide this correction.
Bazett’s and Fridericia’s corrections essentially aim to normalize the QT interval to a heart rate of 60 bpm. The nomenclature (and notation) used for these two corrections is as follows, where RR is the interval between the R wave of one ECG and the R wave of the subsequent ECG:
and
ICH E14 proposed that several values should be reported when presenting the results from a TQT study. These include the following:

![$$ \mathrm{QTcF} = \frac{\mathrm{QT}}{{\mathrm{RR}}^{\raisebox{1ex}{$1$}\!\left/ \!\raisebox{-1ex}{$3$}\right.}}=\frac{\mathrm{QT}}{\sqrt[3]{\mathrm{RR}}} $$](https://i0.wp.com/thoracickey.com/wp-content/uploads/2017/06/A333533_1_En_7_Chapter_Equb.gif?w=960)
The actual (uncorrected) QT interval
Heart rate
QTcB
QTcF
QT corrections of any other type should the researchers wish to employ additional correction factors
However, QTcB is now widely accepted to be an inferior method of correcting for heart rate, and using QTcF is considered appropriate in most situations.
An alternative correction employs linear regression techniques to normalize the QT interval to a heart rate of 60 bpm. ICH E14 discusses a correction calculated in this manner in the Framingham study (see Kannel and Sorlie 1975; Tisdale, et al. 2007). Regression approaches can also be used from other large databases in a similar manner: employing pretreatment data from a study, QT interval measurements are regressed on (1-RR) to obtain estimates of the slope for the following equation:
Another correction methodology employs linear regressions that are calculated for each individual participant in a TQT study: this methodology yields the individual corrected QT or QTcI. Pretreatment QT and heart rate data are used to fit a separate regression for each participant. This means that a slope coefficient is applied to each participant on an individual basis, rather than using the same coefficient in a population-level regression approach. The employment of QTcI has been advocated as the primary endpoint in TQT studies where the drug being tested is known to exert notable influence on heart rate. However, when QTcI is employed as the primary endpoint, it is good practice to report QTcB (for historical reasons) and QTcF as well (Litwin et al. 2008).

7.5 Further Discussion of ICH E14 and the TQT Study
The TQT study was discussed to a certain degree in Chap. 5 so that the related hypothesis-testing statistical methodologies, the union–intersection test and the intersection–union test, could be placed in this context. The explicit objective of the TQT study is to provide an accurate and precise estimate of a drug’s effect on the QTc and, based on this assessment, to guide the extent of ECG monitoring that will be needed in Phase III (Bloomfield 2015). At this point it is appropriate to recap the key points of the TQT study’s traditional design before presenting new material discussing the TQT study in more detail.
As noted at the beginning of this chapter, the first goal of the field of proarrhythmic cardiac safety is to determine to the greatest degree possible during preapproval research whether a noncardiac drug has the propensity to lead to torsades in patients who may be prescribed the drug should it subsequently be approved for marketing. As represented in TQT studies, which are typically conducted in clinical pharmacology units within residential or inpatient medical centers to facilitate 24-h supervision and extensive monitoring of participants’ health status in addition to the collection of the necessary ECG waveforms, QTc prolongation is considered as a biomarker for torsades. Therefore, the purpose of the TQT study is to prospectively exclude an unacceptable degree of drug-induced QTc interval prolongation.
The TQT study is a randomized, placebo- and positive-controlled clinical trial that can adopt a crossover design or a parallel-group design. The traditional four treatment arms are as follows:
A positive control arm, comprising of a drug that is known to increase the QT interval by around 5 msec. This is employed to establish assay sensitivity, i.e., to demonstrate that the methodology employed is able to detect a prolongation of the QTc interval when it is indeed present.
A placebo arm, against which the drug is compared.
The proposed therapeutic dose of the drug.
A supratherapeutic dose of the drug: unless the proposed dose is close to the maximum tolerated dose, the supratherapeutic dose is likely to be several multiples of the proposed dose.
The choice of a crossover design (in which each participant receives all four treatments in a specified sequential order) or a parallel-group design (in which each participant receives only one treatment and which therefore necessitates four times as many participants) should be considered carefully. The primary advantage of the crossover design, which is chosen more often than the parallel design, is that each participant serves as his or her own control, thereby reducing the inter-participant variability for estimates of the drug’s effects on the QTc interval. A direct corollary of this reduction in variability is that a smaller sample size is required for an appropriately statistically powered study. It is advisable to use balanced crossover designs, in which each treatment is administered to some participants prior to every other treatment, to ensure that any crossover effect is balanced. However, crossover trials are typically not recommended when the test drug has a long half-life (and hence requires long washout periods) or when multiple doses are being evaluated: in these instances a parallel-group design may be considered advantageous (Turner and Durham 2009).
TQT studies are typically conducted at some point following completion of single- and multiple-ascending dose (SAD and MAD) studies and others such as renal impairment, hepatic impairment, and drug–drug interaction studies, which help determine the supratherapeutic dose, frequently the most difficult choice in TQT study design (Turner et al. 2015). Optimal TQT study design requires fundamental knowledge of the drug’s clinical pharmacokinetic profile, including estimates of C max and T max, since QTc prolongation must be assessed shortly before, at, and shortly after T max in addition to some later time points: these typically include 24 h after drug administration and additional later time points should the drug have late-appearing metabolites that may themselves have a proarrhythmic influence.
7.5.1 Statistical Analyses Discussed in ICH E14
The statistical details of the intersection–union and union–intersection tests used in the analysis of QTc data following the completion of a TQT study were provided in Chap. 5 since we believe that readers interested in that level of detail should have access to it within this book. That said, our discussions in this chapter are couched in more simple nomenclature.
ICH E14 noted that “The QT/QTc interval data should be presented both as analyses of central tendency (e.g., means, medians) and categorical analyses. Both can provide relevant information on clinical risk assessment” (ICH 2005b). Categorical analyses, which are descriptive in nature and the simpler of the two sets of analyses, are discussed first.
Categorical analyses are based on the number and percentage of participants that meet or exceed a predefined upper limit. Such limits can be stated in the study protocol or the associated statistical analysis plan in terms of absolute QTc interval prolongation values or change from baseline. At the time of the guidance’s release, there was no consensus concerning the best choice of these limits. The guidance therefore suggested that multiple analyses using several predefined limits were a reasonable approach in light of this lack of consensus. For absolute QTc interval data, the guidance suggested providing absolute numbers and percentages of subjects whose QTc intervals exceed 450, 480, and 500 msec. For change-from-baseline QTc interval data, the same information might be provided for increases exceeding 30 msec and those exceeding 60 msec.
Now, consider the second part of the overall analytical strategy, which ultimately focuses upon the mean QTc prolongation induced by a test drug. The first aspect of this part is the demonstration of assay sensitivity. Moxifloxacin, given as a single oral dose of 400 mg, is the typical choice of positive control (other possibilities have been discussed in the literature, e.g., see Taubel et al. 2010). Moxifloxacin is an 8-methoxyquinoline antibacterial with enhanced potency against important gram-positive pathogens, notably Streptococcus pneumoniae (see Burkhardt and Welte 2009; Albertson et al. 2010; Tulkens et al. 2012; Chuchalin et al. 2013; Kuzman et al. 2014). Moxifloxacin-induced QTc effects have been reported in the literature (e.g., see Yan et al. 2010). The pharmacokinetic properties and QTc time-course profile of moxifloxacin are well characterized: the profile includes a rising phase, a peak around 2–4 h postdose, and a tapering phase. Litwin and colleagues (Litwin et al. 2008) cited an average moxifloxacin-induced increase in the QT interval of 5–8 msec: other authors have suggested slightly higher values.
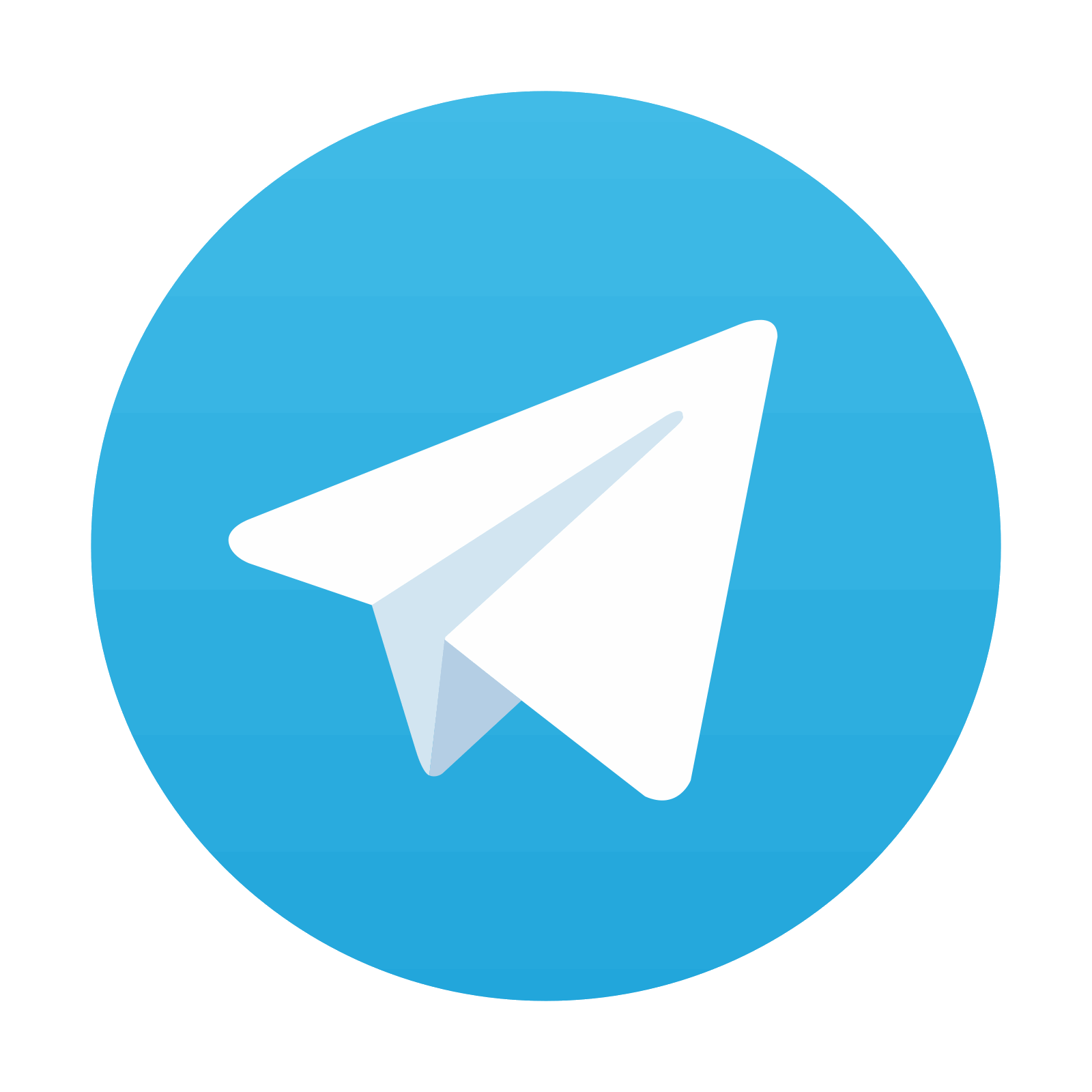
Stay updated, free articles. Join our Telegram channel
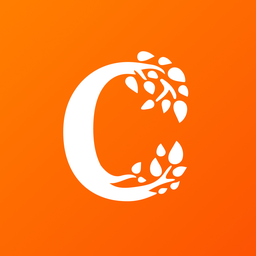
Full access? Get Clinical Tree
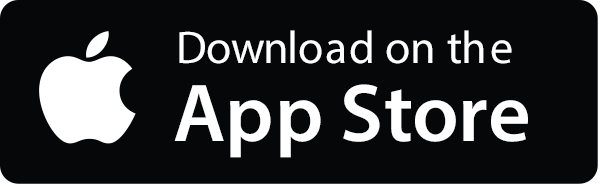
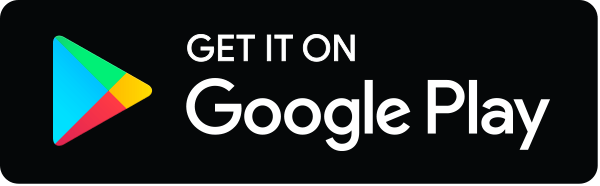