CHAPTER 1 Maintenance, regular turnover, and repair of bone are critically dependent on a sufficient vascular supply and responsive hemodynamics. Bone circulation obviously acts as a conduit to provide nutrients for cell in bone, but in addition, it has an important role in mineral homeostasis, the control of bone turnover, and the response to injury. Any event such as a fracture or surgical intervention to bone poses a challenge due to disruption of the blood supply, and requires an intact vascular response for successful repair. The specific anatomical organization of the vascular system in the skeleton varies from bone to bone, and details have been described.16, 29 There are, however, common underlying principles for the vascular organization in all bones, and these are important for many of the physiological functions of the vascular system. This section, therefore, will provide an overview of the anatomic sources of blood flow to bone, and their hemodynamic interconnections. Following historical accounts by Langer72 and Lexer,74 there is agreement that the blood supply of bone has three main sources of vessels supplying the cortex of long bones:16, 63 the epiphyseal-metaphyseal arteries, the nutrient artery, and the periosteum. Bone marrow is perfused by blood vessels arising from the one or two nutrient arteries which have pierced the bone. In over 90% of the human tibiae examined by Menck et al. there was one nutrient foramen evident, but in a few cases there were two such foramina.92 The nutrient vessels will not solely supply marrow, but cortical bone as well. In the diaphyseal marrow of tubular long bones, the nutrient artery divides into ascending and descending branches and, together with the medullary branches of the metaphyseal arteries, is responsible for the blood supply to the fatty and haemopoietic bone marrow. Lopez-Curto et al. used stereo light-microscopy for three-dimensional examination of the vasculature in the adult dog tibia and showed that the vasculature of the cortex and the medulla did not communicate at an arteriolar or capillary level.80 They concluded that the nutrient artery supplied the marrow and cortex in parallel. The periosteal vascular plexus, the Hunter’s vascular circle, around the metaphysis perforates the cortex in a pattern constant throughout the life of an individual. These arteries divide within the metaphyseal area and anastomose with branches of the nutrient artery.142 Anecdotal evidence suggests that the metaphyseal arteries are capable of sufficiently supplying the diaphysis in long bones with congenitally absent nutrient foramina.16 Following the closure of the epiphysis, intraosseous — in addition to the existing extra-osseous — anastomoses occur between the metaphyseal and epiphyseal arteries. The vascular network thus formed is referred to as the epiphysio-metaphyseal vascular system.99, 138, 140 The arrangement of the venous system of the marrow is different from that of the arterial system. A central venous sinus with a diameter approximately four times that of the nutrient artery, but with a thinner wall, runs medullo-central the full length of the diaphysis.16 The nutrient vein branches off the central venous sinus as well as other emissary vessels and traverses the cortex.15, 80 The periosteum forms a thin surrounding layer of soft tissue which envelopes bone. It is most distinct at the level of the diaphysis and particularly well established in the young organism. A fracture of the juvenile skeleton is often referred to as a “greenstick” fracture when the periosteal layer has stayed intact. The periosteum forms the interconnection of cortical bone with the musculature and connective tissue. The periosteum consists ultrastructurally of three layers of tissue: close to bone the cambium layer contains osteogenic cells, in the midzone a highly vascular layer contributes to the blood supply of the cortical bone, and in the periphery there is a dense layer of collagen bundles and fibers.45 The periosteum is responsive to injury two-fold: its osteogenic layer forms the peripheral callus in fracture healing and its vascularity acts as reserve supply should the medullary system fail. Menck et al. have given a detailed account of the anatomy of the arterial supply to the periosteum of the human tibia.92 They dissected 30 legs after the injection of Berlin Blue Gelatine and found crucially that the distal diaphysis is supplied exclusively by semicircular branches of the anterior tibial artery. In contrast, the proximal diaphysis is supplied by periosteal branches of the anterior and posterior tibial artery. As clinically observed, it is in the distal tibia that fracture healing is often impaired. The vasculature in cortical bone utilizes the spaces provided by the longitudinal Haversian system and the transverse Volkmann canals, although the causative relationship is probably the reverse. A description of the structure of compact bone will outline the arrangement of the angioarchitecture of bone. Cortical bone is structured in a highly organized fashion and intracortical vessels play a central role. The osteon is a term often used interchangeably with the Haversian system and should be preferred if the structure is addressed. The osteon constitutes the basic morphological unit of most of the compact (cortical) bone, together with the circumferential and interstitial lamellae that are deposited at the periosteal and endosteal surfaces.23 The vessels in the osteons were found to be continuous with vessels coursing in endosteal lamellae, most of which pierce the subendosteal lamellae. The network of osteons follows a spiral around the axis of the bone. A Volkmann canal — a term for a vascular channel which is not surrounded by concentric lamellae of bone — forms a radial connecting canal passing through circumferential lamellae.26 Cooper et al. performed electronmicroscopic studies on the Haversian system. Within each osteon they found one or two vessels with the ultrastructure of capillaries.26 The vessels are lined by endothelial cells and often connected by a special single-layered membrane. Immediately adjacent, but not forming a complete ring, pericytes were seen. The basement membrane splits to include the pericytes.34 Usually there were unmyelinated and sometimes myelinated nerve fibers 5–9 µm in diameter seen.26 Mature osteons gradually transform into resorption spaces and new osteons will be formed in such spaces. All osteons are demarcated from their neighbors by a jagged-edged cement line which may mark the limit of bone erosion prior to the formation of an osteon.23 Similar basophilic lines also occur in the absence of erosion when bony growth is interrupted and then resumed (resting lines). Canaliculi may sometimes pass through the cement lines, thus providing a route for exchange between interstitial bone and osteons.5 Most individual blocks of interstitial lamellae were structurally continuous with osteons, either distally or proximally, thus providing an axial pathway for diffusion of fluid through compact bone between the periosteal and endosteal surfaces.23 Based on the anatomical basis of the cortical structure is the concept of a vascular connection between the different sources. Thus, a compensatory response between systems becomes possible if one system fails. There has been a considerable debate and opponents have denied intracortical anastomoses between the medullary and periosteal vascular systems.16 However, a number of workers have described communications between the periosteal and medullary sources of cortical blood flow, as early as Drinker & Drinker.40 Using the Spalteholz method of clearance and stereo-lightmicroscopy, Trias and Féry described the Haversian and Volkmann canals and arrived at a network-like model of the vasculature of the canine femur.137 Lopez-Curto et al. observed transcortical periosteal-medullary arterioles, conduit vessels, which form anastomoses between branches of the nutrient artery and the periosteal vessels in the adult dog tibiae following perfusion with Microfil.80 Skawina et al. support with their work using corrosion casting to investigate the human fetal long bone the existence of intracortical anastomoses.128 Crock concurs showing illustrations of those in his atlas.29 The descriptive work of the vascular structure of cortical bone has been marred by methodological difficulties. Most injection studies use the nutrient artery as a route to inject the material to visualize the arterial system, thus demonstrating solely the centrifugal route of flow. Often the periosteum is removed from the illustrated specimens. Of importance is the concept of sufficient intracortical vascular connections, anastomosing between the medullary, metaphyseal, and periosteal sources of blood flow. As suggested by Trias and Féry137 the existence of intracortical anastomoses gives the anatomical basis for blood flow direction to change according to the prevailing physiological conditions.139 Techniques for the measurement of blood flow to the skeleton are described in later chapters of this book. Bone poses specific problems for the measurement of blood flow: (1) there are 206 separate bones in the skeleton; (2) as discussed above, each bone has multiple arterial inputs and venous outflows; (3) each bone is heterogeneous, comprising varying proportions of cortical bone, cancellous bone, and marrow (both hematopoietic and fatty). Because of this heterogeneity of the tissue, it is important to specify precisely the region of bone that is being measured. These issues probably account for some of the variation in values of bone blood flow reported in the literature. It is now generally accepted that approximately 5% of the cardiac output goes to the skeleton.123 Within this overall figure, there is wide variation in absolute blood flow values between different bones132 as well as between cortical bone, trabecular bone, bone marrow, and periosteum. Typical values of blood flow to these tissues are shown in Table 1.1. Trabecular bone has a higher rate of turnover than cortical bone, as well as containing marrow, and this is reflected in a higher blood flow in trabecular bone. The periosteum also has significantly higher absolute blood flow than cortical bone,118 reflecting its higher cellularity. Table 1.1. Typical values of bone and periosteal blood flow. Source: Reichert ILH (2000).118 Although cortical bone appears relatively poorly perfused, the cellular volume in cortical bone is also low (see section 1.3.3). This has led to the suggestion that cortical bone perfusion is higher than that required to meet the metabolic needs of the tissue, and that the magnitude of bone blood flow is determined by requirements for bone to act as a buffer in mineral exchange for calcium homeostasis.116 It is interesting to do some simple arithmetic, combining these fluid space data with blood flow data presented earlier. Although blood flow to cortical bone is relatively low in absolute terms, it is very high if expressed in terms of cellular mass of the tissue. This is also consistent with the observations of active control of mineral ion transport discussed later. Several means of examining blood flow to and in the diaphyseal cortex have been used to determine the normal blood flow direction. Brånemark used intravital microscopy to directly observe the blood flow in rabbit fibulae and found that the capillaries coming from the marrow arterioles enter the Haversian canals to supply the endosteal diaphysis only.12 The capillaries then return to the marrow to empty into the sinusoids. Since only the endosteal diaphysis is centrifugally supplied, the remainder of the diaphyseal cortex must be supplied centripetally. Occluding a given source of blood flow by ligation or obliteration followed by blood flow measurement will give an estimate of the contribution of this source. Such experiments aiming for quantified flow partition have also indicated that blood flow is centrifugal. Kelly used the hydrogen washout technique to measure blood flow in the canine tibia and reported that 71% of nutrient artery flow supplies the cortex and 30% the marrow.64 Cortical blood flow has been measured using the indicator fractionation technique with radio-labeled microspheres which is widely regarded as the gold standard method. Values of 2–5ml/min/100g of blood flow have been measured in the diaphyseal cortex of canine long bone.75 Tothill and McPherson used this technique and measured regional blood flow in cortical bone with radiolabeled microspheres pre- and post-ligation of the nutrient artery.136 They reported that the nutrient artery contributes 70% of total diaphyseal cortical flow. Willans obtained lower figures of 62% contribution by the nutrient artery to total cortical flow in the canine tibial diaphysis.153 Kowalski et al. performed a similar study measuring the contribution of the periosteum to cortical bone, in that they scraped the periosteum off the diaphysis, and found a reduction of cortical flux by 20% using laser Doppler flowmetry.69 Measurements of the intramedullary pressure (IMP) provide the most conclusive evidence for normal centrifugal flow. Fluid moves down the pressure gradient. Pressure in the marrow cavity has been measured in experimental animals and human subjects by a number of investigators and found to be higher than the surrounding soft tissues. The most meticulous measurement has been performed by Wilkes and Vischer,152 who were able to remove the cortical bone to expose the endosteal membrane and to place the pressure transducer directly on this tissue layer. Thus they maintained the entity of the medullary cavity whilst performing the measurement. IMP is reported to be between 45mmHg and 60mmHg in the human.16 The blood pressure in the nutrient artery must be correspondingly high for flow to access the medullary cavity. Tøndevold measured in dogs that arterial inflow into the medullary cavity stopped when the arterial blood pressure fell below 80mmHg.135 Extra-osseous capillaries in surrounding soft tissues measure a pressure in the range of 12 to 15mmHg, which is lower than pressure in the medullary cavity.16 Thus, the vascular driving pressure is centrifugal across the cortex from marrow to periosteum and blood flow direction is normally centrifugal. Based on the structural as well vascular connectivity between the vascular systems of cortical bone it is reasonable to suggest a compensatory response if that is required by the haemodynamic situation. Trueta, a surgeon as well as researcher, proposed the following suggestion, based on clinical observation: “… if one vascular system breaks down another takes over.”139 The capacity of the cortical vasculature to respond to afferent breakdown has been studied by occluding one or two of the three afferent systems and examining the cortical viability using a variety of histological, angiographic, and quantitative methods. Clinically relevant is the investigation on cortical and periosteal blood flow following intramedullary reaming as a surgical procedure prior to intramedullary nailing, which occludes the nutrient artery and occludes the medullary system. The indicator fractionation technique has been extended to measure blood flow in the periosteum in a large animal model to establish the effect of reaming on blood flow in the tibia. The results show a six-fold increase in periosteal blood flow 30 minutes after intramedullary reaming and no change in net cortical blood flow.117 This indicates an acute compensation for the occlusion of the medullary vascular system by the periosteum. Capillary structure is conventionally characterized as either continuous or fenestrated, or sinusoids. Capillaries in cortical bone are described as continuous, and microcirculatory vessels in bone marrow are commonly characterised as sinusoids. Beyond this gross classification of capillary morphology, it is now appreciated that vascular endothelial cells have tissue-specific characteristics dependent on cell surface markers. In bone, endothelial cells exhibit enhanced surface expression of the chemotractant SDF-1, and have characteristic heparin sulphate proteoglycan and adhesion molecule profiles.59 However, not only does bone differ from other tissuesbut there is also important variation within bones. Recently, detailed characterisation of cell surface markers of capillaries has shown heterogeneity of surface markers even within the same bone. In mouse tibiae and femora, staining vessels for CD31 and endomucin (emcn) has indicated that there are two types of vessels — those staining strongly for CD31 and emcn (CD31hiEmcnhi — type H) and those staining weakly for the same markers (CD31loEmcnlo — type L).71 Type H vessels were primarily observed near the metaphysis, and osteoprogenitor cells were selectively positioned around type H vessels, even though type H vessels only make up around 1.8% of the vessels. It was also shown that the proportion of type H vessels decreases with age, suggesting that these type H vessels may be implicated in bone changes observed in osteoporosis. The heterogeneity was not observed in most other tissues, and apart from bone, type H vessels were only found elsewhere in the liver.71 Transcapillary transport can be classified as flow-limited or diffusion-limited. In the former, transcapillary transport is very rapid, and the rate of delivery of solutes to tissue is limited by the flow to the tissue; in the latter, the rate limiting factor is transcapillary exchange rather than flow to the tissue. It has been shown that transcapillary transport is diffusion-limited, rather than flow-limited. Studies of capillary permeability in bone were developed by Patrick Kelly at the Mayo Clinic, who adapted the outflow dilution technique applied to other organs for use in bone Perfusing the tibial nutrient artery at a controlled rate, radioactive traces of a range of molecular weights and/or chemical properties would be injected as a bolus into the nutrient artery, and passage of the tracer through bone could be studied by collecting blood from the femoral vein. Such studies characterized the transcapillary exchange as being passive and diffusion-limited.73 It was also possible to use this technique to investigate the kinetics of tracer movement beyond the capillary wall,85 using, for example, calcium and potassium analogues to investigate extravascular transport rates. Although “dry as a bone” is a common expression, bone has a significant fluid space. This fluid space is portioned between vascular, cellular, and extravascular extracellular. In mature cortical bone, the fluid space amounts to about 0.287ml/ml bone by volume of the cortex.97 The distribution of bone fluid between vascular, cellular, and interstitial (extravascular and extracellular) spaces is illustrated in Fig. 1.1. Vascular space comprises 6.3% of the total fluid space, and the cellular and interstitial spaces comprise 25.1% and 68.6%, respectively. These values are for mature cortical bone; fluid spaces are larger in immature bone.108 For a while, it was suggested that there was a discrete bone extracellular (BECF) fluid space, which was separated from the perivascular fluid space in the Haversian canals.17, 101 It was originally proposed that there was a membrane delineating the bone fluid space, identified as a potassium-rich bone fluid space.103 However, such a discrete potassium-rich space has not been found, and the potassium content of bone can probably be accounted for by the cellular space.108 It is possible, though, that cells can regulate the composition of the pericellular extravascular fluid. Although it has been difficult to investigate the composition of a specific BECF, other experiments indicate that an active transport system may contribute to short-term plasma calcium homeostasis without a contribution from bone remodeling. The rates of calcium exchange at the bone-plasma interface have been estimated to be about 50 to 100mM Ca per day. This is about ten times higher than the daily flux due to bone remodeling, and three times than the daily flux in the kidney under PTH control.115 Two experiments have aimed specifically at measuring any active component in mineral influx and efflux.86, 89 McCarthy and Hughes used the outflow dilution technique described above in the perfused tibia, and compared the effect of potassium cyanide in the perfusate on Sr86 (Ca analogue) and potassium (intracellular ion) outflow; Marenzana et al. measured real time Ca++ efflux from mouse weanling metatarsal bones, using the scanning ion-selective electrode technique (SIET).91 Although very different in approach, both experiments showed passive influx of calcium into bone, but a small active component of efflux from bone. The exact mechanism of active control is uncertain, but the results are consistent with a pump-leak mechanism, probably under the control of an osteocyte-bone lining cell syncytium. Another issue concerning extravascular transport in bone, irrespective of active control of calcium fluxes in ion exchange, is the nature of movement through the BECF, particularly for larger protein molecules.18 Typically, an osteon within cortical bone is approximately 200µm in diameter. This means that osteocytes within the bone matrix can be up to 100µm from a capillary. Cortical bone has a very extensive network of channels allowing communication between plasma and bone matrix. Canaliculi, approximately 0.1µm in diameter, radiate from the Haversian canal and form a complex network connecting osteocytes with the Haversian canal and other osteocytes. Radiating from the canaliculi are submicroscopic interfibrillar spaces of bone matrix. The extent of these networks is so great that it has been estimated that the surface area of the canalicular system is approximately 250mm2/mm3, and the interfibrillar spaces represent an exchange area of the order of 35,000mm2/mm3. It has been speculated that, because distances between osteocytes and capillaries are relatively large in microvascular terms, diffusion may not be sufficient to supply these cells and that a more rapid turnover of interstitial fluid in bone aids the process of cell nutrition. The role of IMP in determining the direction of blood flow in cortical bone has been discussed above; similarly, IMP is thought to have a role in fluid movement through interstitial space. Experimental evidence suggesting that convective flow may contribute to the nutrient of osteocytes comes mainly from studies on the movement of labeled protein molecules (such as albumin, thorotrast, or ferritin) through both unloaded and loaded bone.33, 96, 113 One problem with this type of experiment is to confirm that there is no movement of these tracers post-mortem during processing of the tissues; another problem is that bones are processed at specific times after injection of the tracer, providing isolated snapshots of tracer movement. Using mathematical modeling, it was argued that mechanical loading would enhance perfusion and aid nutrition of osteocytes,107 but Yang argued that the nutritional requirements of osteocytes were unknown, but presumably relatively low, and that mechanical load may not be required.90 Enhancement of extravascular transport resulting from mechanical loading has been demonstrated.134 Recently the technique of fluorescence bleaching after photobleaching (FRAP) has enabled dynamic measurement of solute movement in extravascular fluid in the murine tibia.78, 111, 150 Using sodium fluorescein (376 Da) as a probe, no effect of vascular pressure on transport into bone was observed, and theoretical model for small (376 Da) and large (43,000 Da) molecules indicated that vascular pressure-driven convection was at least an order of magnitude smaller than diffusion in transport of solutes through bone interstitial fluid.78 In contrast, mechanical loading inducing about 400–500 microstrain enhanced the transport of sodium fluorescein (+31%) and parvalbumin (+50%).111, 113, 150 In addition to contributing to solute movement through bone interstitial fluid space, mechanical loading also provides a mechano-transduction signal mediated by shear forces generated by load-induced transient fluid movement acting on osteocytes.67 Details of the osteocyte response to load are beyond the scope of this chapter, but among factors released is vascular endothelial growth factor (VEGF), which not only stimulates blood vessel formation, but also stimulates osteoblastogenesis.21 Although the role of vascular intramedullary pressure on fluid movement through bone is equivocal, there are many instances in which there is an association between vascular pressure and bone density. The response of bone to load is site-specific, related to local interstitial fluid pressure.141 In environments in which the distribution of cardiovascular pressures is changed (such as microgravity), bone density changes correlate with pressure changes, and there is apparent increase in bone density in regions with positive changes in cardiovascular pressures.92 Rat tail suspension is an experimental approach to mimic the effects of microgravity, and bone density is decreased in the lower limb. Ligation of the femoral vein reduces the bone loss associated with tail suspension;7 ligation also produced a significant increase in intramedullary pressure, and it was proposed that this increase in pressure resulted in enhanced interstitial fluid flow through bone, which mediated the bone response. Another experimental study ligating the popliteal artery in the caprine tibia caused intraosseous hypertension and resulted in significant increases in periosteal, endocortical, and trabecular bone formation.151 However, theoretical studies suggested that the pressure changes were insufficient to have much effect of fluid flow, and that pressurization of the periosteum may be the causal link.149 Dynamic pressure application has been shown to be effective in modifying bone mass. In a rat tail suspension model, dynamic hydraulic stimulation at a frequency of 2 Hz for 20 minutes a day was able to induce peak-to-peak variations of IMP of either 10 or 15mmHg.57 This intervention was found to eliminate the loss of bone volume fraction in the tibia caused by tail suspension. It was argued that the hydraulic stimulation produced direct muscle coupling, temporarily occluding vessels in bone, and generating an A-V pressure gradient driving fluid flow.57 There are also clinical examples of an association of vascular pressures and bone density. For example, Arnoldi et al. observed bone density changes in patients with intra-calcaneal hypertension.4 It has also been shown that hypertension is associated with higher bone mineral density measurements for both women and men.53 Atherosclerosis has been shown to be associated with reduced bone density.100 Although these observations show an association between vascular pressures and bone density, it is not clear at the moment as to the nature of this association. An alternative explanation for this association could be that endothelin signaling could serve as a nexus for the convergence of vascular and skeletal modeling.149 Values of bone blood flow quoted above are typical values. However, as in most tissues, neural, humoral, and metabolic parameters all contribute to the regulation of blood flow and vascular resistance in bone. Bone and periosteum are innervated by both sympathetic and sensory nerves.8, 27, 59, 87, 126 Several different staining techniques have been used to study the distribution of nerve fibers in bone. Most nerves are perivascular, and the periosteum, metaphysis, and epiphysis are more densely innervated than cortical bone. As well as noradrenergic sympathetic fibers, peptidergic nerves containing substance P (SP), calcitonin gene-related peptide (CGRP), vasoactive intestinal peptide (VIP), and neuropeptide Y have also been described.9 Many SP- and CGRP-immunoreactive fibers were observed in association with blood vessels in both periosteum and cortical bone. During fracture repair, periosteal CGRP-containing nerve fibers are very actively proliferating elements, and it has been suggested that vascular control and stimulation of angiogenesis are major functions of CGRP during the fracture healing process.59 The most precise physiological measurements of vascular reactivity of bone have been performed in experimental preparations that perfuse the nutrient supply of a long bone at a constant flow rate, and in which perfusion pressure is continuously monitored to investigate changes in vascular resistance in response to the injection of vasoactive agents into the perfusion circuit.14, 28, 30, 38, 39, 51, 91 An alternative approach has been to study isolated resistance arteries from bone.37, 81 In general, it has been shown that the vasculature in bone responds to most vasoconstrictor and vasodilator substances. However, comparing the vasoreactive responses in bone with those in other tissues, it has been proposed that bone is relatively hypersensitive to vasoconstrictors, and relatively hyposensitive to vasodilators.14 Responsiveness to α– and β-adrenergic agonists has been observed, as well as the presence of prostaglandin H2/thromboxane A2 receptors. Table 1.2 summarizes some of the major observations of vascular reactivity in bone. Qualitatively, the response in bone is, in general, similar to other vascular beds, even if quantitatively responses can be very different. The most surprising observation is the action of isoproterenol, which has a vasodilator effect in most vascular beds but cause significant constriction when injected into bone.14 Table 1.2. Summary of effects of vasoactive agents on bone.
THE PHYSIOLOGY OF BONE CIRCULATION
1.1 Introduction
1.2 Organization of the Vascular System in Bone
1.2.1 Medullary circulation
1.2.1.1 Parallel supply of marrow and cortex by the nutrient artery
1.2.2 Metaphyseal circulation
1.2.3 Venous system
1.2.4 Periosteal circulation
1.2.5 Structure and blood supply of the diaphyseal cortex
1.2.5.1 Ultrastructure of Haversian systems
1.2.5.2 Intracortical anastomoses
1.3 Distribution of Bone Blood Flow
Blood Flow (ml/min/100g)
Cortical Bone
1
Trabecular Bone
5
Cortical Marrow
1
Periosteum
5
1.3.1 Hemodynamics of flow direction in cortical bone
1.3.1.1 Quantitative flow partition experiments
1.3.2 Intramedullary pressure
1.3.3 Compensatory vascular responses in cortical bone
1.4 Bone Microcirculation
1.4.1 Capillary ultrastructure
1.4.1.1 Capillary characterization by surface markers
1.4.2 Transcapillary exchange
1.4.3 Bone fluid spaces
1.4.4 Ion exchange in bone
1.4.5 Extravascular solute transport
1.4.6 Fluid pressure and bone density
1.5 Control of Bone Blood Flow
1.5.1 Perivascular innervation
1.5.2 Vascular reactivity
Agent
Vascular resistance
References
Acetylcholine
↓
Driessens & Vanhoutte (1979)
Adenosine
↓
Gross et al. (1979)
Adrenaline
↑
Drinker & Drinker (1916)
Bradykinin
↓
Lundgaard et al. (1997)
Calcitonin
↑
Driessens & Vanhoutte (1981)
CGRP
↓
Lundgaard et al. (1997)
Endothelin
↑
Brinker et al. (1990); Briggs et al. (1998); Drescher et al. (2006)
Isoproterenol
↑
Brinker et al. (1990)
Methoxamine
↑
Brinker et al. (1990)
Nitric oxide
↑
Lundgaard et al. (1996); McCarthy et al. (1997)
Noradrenaline
↑
Gross et al. (1979); Driessens & Vanhoutte (1979); Lundgaard et al. (1996)
Prazosin
↓
Dean et al. 1992
Propranolol
↓
Dean et al. 1992
Rauwolszin
↓
Dean et al. 1992
Substance P
↓
Lundgaard et al. (1997)
U46619
↑
Brinker et al. (1990)
VIP
↓
Lundgaard et al. (1997)
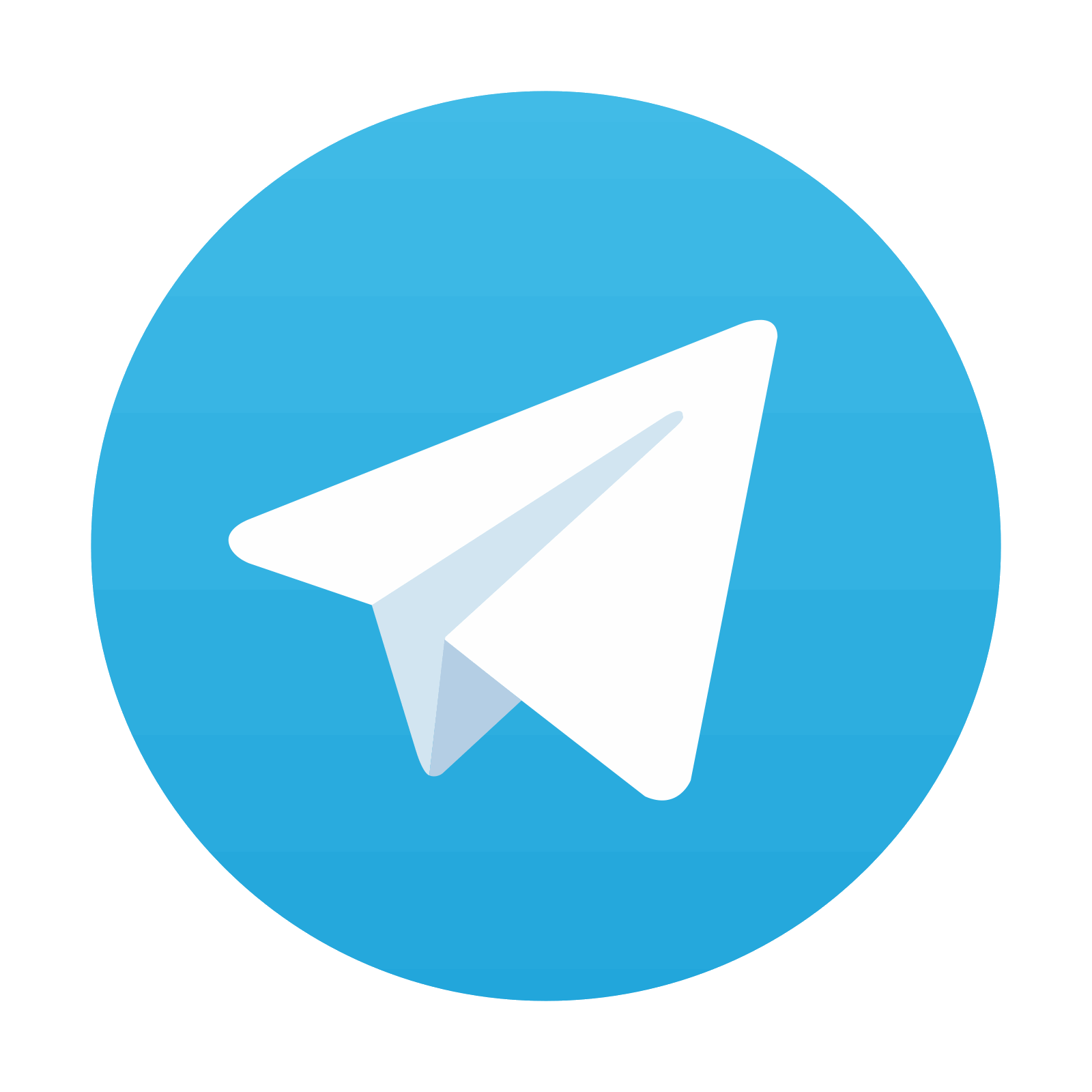
Stay updated, free articles. Join our Telegram channel
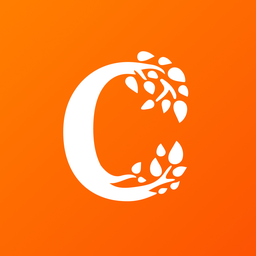
Full access? Get Clinical Tree
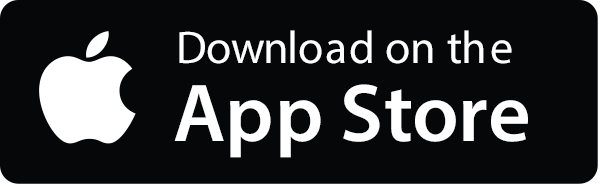
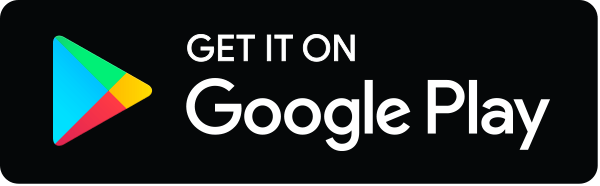