Fig. 13.1
Hemodynamic stress causes morphological changes of the heart and the central vessels which are initially adaptive (upper box). These factors affect the fate of the myocardial cells (lower box)
Definition of Chronic Right Ventricular Failure
Chronic right heart failure, like left ventricular failure, is often defined as a syndrome [9] and graded as “risk of developing RVF, asymptomatic RV dysfunction, symptomatic RVF and end-stage RVF” [9]. The 2009 ACCF/AHA Heart Failure guidelines emphasize “that heart failure is not equivalent to cardiomyopathy or cardiac dysfunction.” Instead, heart failure is defined as a clinical syndrome that is characterized by specific symptoms (dyspnea and fatigue) and signs (edema, gallop rhythm, rales) on physical examination. “Heart failure is a symptomatic disorder and is not diagnosed by a single test” and “the mechanisms responsible for the exercise intolerance of patients with chronic heart failure have not been defined clearly” [9]. While it is not difficult to recognize the signs and symptoms of severe RVF, it remains difficult to define RV dysfunction and to predict when an individual patient transits from compensated to decompensated RVF. Clinicians are discussing whether there are different RV phenotypes [10, 11] and whether there are variables that can be measured to determine whether patients have a “failure prone” or a “failure resistant” RV. The flow diagram (Fig. 13.1) proposes that neurohormonal overdrive as well as inadequate RV hypertrophy may lead to RVF. We believe that RV dysfunction exists when the patient’s dyspnea can be explained hemodynamically by an elevation of the right atrial pressure and/or a lower than normal cardiac index at rest. Perhaps the condition of “at risk of developing RVF” describes the asymptomatic patients which demonstrate mild abnormalities when examined by echocardiography (see Chap. 10) or cardiac MRI (see Chap. 12). We wonder whether a mildly elevated BNP level in patients with PAH may be a reliable marker of early RVF, however, it may be wishful to think that BNP alone will be able to explain and predict RV dysfunction in each individual case, and if so, this finding would violate the “no single diagnostic test” rule.
Mechanical Concepts of Right Heart Failure
In patients with pulmonary hypertension, the increase in pulmonary vascular resistance impacts the RV. Analogous to chronic obstructive pulmonary diseases (COPD), which are defined by an increased resistance to airflow, the right ventricle is challenged when working against an increased resistance to blood flow. The increase in resistance can be in close proximity to the RV outflow tract as in the setting of pulmonary artery stenosis or central pulmonary artery blood clots and modeled by main pulmonary artery banding (PAB), however, in patients with PAH the structural changes occur in nearly all of the small pulmonary arteries, accounting for the resistance that the RV pumps against. A second component that changes the response of the RV to increased blood flow resistance is the quality or behavior of the Windkessel of the large central pulmonary vessels [12]. Briefly, the arterial side of the lung circulation consists of the central, large capacitance vessels and the small precapillary resistance vessels. The capacitance vessels or conduit arteries can expand and decrease the flow wave energy that is transmitted to the resistance vessels and capillaries. In pulmonary hypertension the conduit arteries become stiff and less compliant. As the pressure within the RV cavity rises, so does the RV wall stress. Wall stress is inversely proportional to wall thickness. A robustly muscular RV, as in patients with Eisenmenger physiology, experiences less severe wall stress than a less muscularized “stretched” RV. Increased wall stress will also compromise the perfusion of the RV muscle. The schematic below (Fig. 13.1) integrates these various failure components. This schematic entails two causality concepts that are still hypothetical: (1) that RV stress and strain lead to neurohormonal activation and (2) that factors which we designate as “intrinsic to the myocardium” are of pathogenetic importance, and thus contribute to RV failure development and/or progression.
It has been recognized for some time that the RV of patients with Eisenmenger physiology does not easily fail. Again, hypothetically, the “Eisenmenger RV” is as muscular as the LV and is characterized by a generous vessel supply. H.A. Zimmerman, more than half a century ago, performing postmortem coronary angiography, demonstrated an increase in the number of small coronary branches in the right and left ventricle, “so much so in the right ventricle as to approach in number the ramifications of the left ventricle” (Fig. 13.2) [13]. We conclude that a robustly muscular and well-vascularized RV is not ischemic and subject to less wall stress. RV hypertrophy per se appears, indeed, not to correlate with mortality [14]. However, in PAH patients blood flow per gram of RV hypertrophy and the mean systolic/diastolic flow ratio in the right coronary artery decline with rising RV systolic pressure [15] and with increasing RV mass (Fig. 13.3). In addition, right and left ventricular myocardial perfusion reserves correlate with right ventricular function [16]. Thus the RV in severe PAH is likely ischemic, but the “Eisenmenger RV” is not. As there is a strong inverse correlation between the RVSP and mean right coronary artery blood flow, stretch/strain of the RV may be an important component limiting coronary perfusion. Quaife et al. estimated the relative RV wall stress in idiopathic PAH patients and found that significant RV hypertrophy tended to lower the RV wall stress. Neither RV end-diastolic volume nor cardiac output correlated with RV wall stress [17] (Fig. 13.4). High plasma levels of ANP and BNP may be signals which emanate from a stretched right atrium and ventricle—relatively independent of the cardiac output. Indeed Sachdev et al. reported that high RV free wall strain was associated with higher BNP levels [18] (Fig. 13.5).





Fig. 13.2
Postmortem angiogram of the coronary vessels. There is an impressive increase in the number of vessels in the right ventricle of the Eisenmenger heart (right) when compared to a normal heart (left). This image has been first published by H.A. Zimmerman ([12], reproduced with permission)

Fig. 13.3
Relationship between right coronary artery blood flow and RVSP and mass of the right ventricle (RV). Right coronary artery blood flow decreases as the RVSP or the mass of the RV increases (van Wolferen, [13], reproduced with permission)

Fig. 13.4
The right ventricle-free wall stress (calculated from cardiac MRI variables) shows a poor correlation with either cardiac output (CO) (a) or the end-diastolic volume of the right ventricle (RVEDV) (b), (Quaife et al. [16] reproduced with permission)

Fig. 13.5
The right ventricular wall strain relates to the functional class of patients with pulmonary arterial hypertension (left) and to the plasma level of the BNP (right) (Sachdev et al. [17] reproduced with permission)
The Importance of Right Ventricular Hypertrophy and Fibrosis
Cardiac Hypertrophy
Hypertrophy of the RV is frequently discussed in the greater context of “heart form and function” [19–23] and the statement which generally applies to the left ventricle: “hypertrophy is mostly bad.” Coghlan and Hoffman [19] compared the round amphibian (Romanesque) LV with the elliptical (Gothic) human and the “extreme Gothic” LV of the giraffe which is capable of generating pressures of 300 mmHg. The magnitude of pump pressure that can be generated and sustained is determined by the form and the quality of the muscle. In contrast to the LV where increased afterload is met with a percent increase in the LVSP (always less than twofold), the increased pulmonary vascular resistance in PAH leads to a four- to fivefold increase in the RVSP, and in order to adapt to this high pressure demands, a robust RV hypertrophic response. The time course of right ventricular hypertrophy development has been recently reported in cats after pulmonary artery banding [24]. As shown in Fig. 13.6, there is an exponential rise in the RV mass during the first 3 weeks after PAB; thereafter, RV mass reaches a plateau. The authors were interested in myocardial collagen synthesis and showed that the collagen volume fraction also increased, but lagged behind and climbed further as the RV end-diastolic pressure became elevated. They went on to investigate fibroblasts from the RV early and late in the development of RVH and concluded that the collagen deposition by fibroblasts is increased in the hypertrophic RV [24].


Fig. 13.6
Change of the pressure–flow relationship following the RV outflow obstruction following pulmonary artery banding (PAB) (left). Increase of right ventricular mass after PAB in cats and collagen amount expressed as a fraction of the tissue volume (right) (Baicu et al. [23] reproduced with permission)
While many physiologic concepts apply to both the LV and RV, it is conceivable that chamber-specific categorical differences in the programs which govern myocardial hypertrophy and fibrosis exist, given the difference in chamber-specific gene expression patterns [25–27]. In hypertrophic cardiomyopathy, mutations of a number of sarcomeric protein genes have been identified of which mutations of the β-myosin heavy chain (β-MHC) gene were the first to be reported [28]; other mutated genes are α-tropomyosin and titin [28]. In left ventricular hypertrophy, the RV-free wall is equally hypertrophic and it is of interest that patients with hypertrophic cardiomyopathy usually do not develop heart failure. Transcription factors which are important during cardiac development like MEF2, GATA4, NFAT, and myocardin have been implicated as regulators of the hypertrophy program. Mechanisms leading to left ventricular hypertrophy are complex and continue to be discovered in genetically modified rodent models [29–33]. There are positive and negative regulators of cardiac hypertrophy, for example, cGMP/PKG represses hypertrophy via antagonism of ERK1/2 signaling [29]. Protein expression changes in the pressure-overloaded RV have been reported and reflect a shift of energy metabolism towards glycolysis and an upregulated expression of phosphorylated HSP-27, a chaperone that plays a role in proteolysis [34]. The protein expression changes in these PAB experiments have been interpreted as a reflection of an adaptive RV response to pressure overload and negative regulators of hypertrophy may become targets for therapy.
Recent experimental studies have investigated histone deacetylase (HDAC) inhibitors in PAB and chronic hypoxia models [35, 36] and the Sugen/hypoxia model of RV failure [37]. The roles of the different HDAC isozymes are complex (Table 13.1) and the topic of cardiac HDACs in hypertrophy and heart failure has been recently reviewed [38]. HDAC inhibitors frequently induce apoptosis [39] and several HDACs are involved in autophagy [40] (see below). Importantly, HDAC inhibitors attenuate cardiac hypertrophy by suppression of autophagy.
The mechanism of transition from compensated hypertrophy to failure appears to be fundamentally a matter of balance between cell survival and death (see below). If the RV hypertrophy in PAH is initially compensatory, then factors promoting growth and preserve the integrity of the microvessels balance cardiomyocyte hypertrophy and heart tissue blood flow. Impaired expression of factors promoting myocardial angiogenic growth and maintenance is likely a hallmark of this transition as discussed in greater detail below. Here it is only mentioned that myocyte-specific deletion of the transcription factor GATA4 in adult mouse hearts decreased VEGF-A expression and capillary density [41].
The molecular events which underlie the transition of the pressure overloaded from compensated RV to failure have not yet been a focus of in-depth explorations and we mostly rely on data that characterize this transition in the LV. For example, Ling et al. documented in KO mice that CaMKIIδ deficiency ameliorated chamber dilatation, apoptosis, and LV dysfunction after TAC [42]. Depre et al. described a cardiac cell survival program which is induced by transient ischemia [43]. This survival gene set includes PAI-1 and PAI-2, ANP, CTGF, and elongation-factor 1α and 2, as well as heat-shock protein, hsp-27.
Cardiac Fibrosis
As mentioned, RV fibrosis due to pressure overload lags behind after the increase in RV mass [24]. Cardiac fibrosis is a hallmark of maladaptive hypertrophy and is characterized by increased deposition of the fibrillar collagens type I and III [44]. The normal heart muscle contains about 50 % fibroblasts, and elegant studies by Takeda et al. have provided evidence that fibroblasts are essential for the adaptive response of the heart to pressure overload. They showed that the transcription factor KLF5 transactivates IGF-1 in fibroblasts and that the secreted protein induced cardiomyocyte growth in a paracrine fashion [45].
Human autopsy studies have shown that the degree of fibrosis correlates with the mass of the LV up to a weight of 250 g, after that there is a plateau of fibrosis and no correlation [44]. Fibrosis may impair the electrical coupling between cardiomyocytes and can reduce the capillary density and thus influence myocyte metabolism. There is evidence for locally produced TGFβ, PDGF, and a role of endothelin and catecholamines in cardiac fibrosis [44]. TGFβ1 is a critical regulator of extracellular matrix metabolism and TGF-β1 levels increase during the development of pressure-overloaded LV hypertrophy [44]. Higher plasma levels of TGFβ1 were associated with an increased risk of incident heart failure in older adults [44]. Although there is no direct evidence for an important causal role of fibrosis in the development of human heart failure, experimental studies suggest that fibrosis is not merely a marker of heart failure. One of the downstream targets of TGF-β is CTGF which is highly expressed in the failing RV from animals with severe PAH [27]. CTGF is essential for the TGF-β-induced collagen synthesis, and CTGF may also play a role in the transformation of mesenchymal stem cells into fibroblasts [46]. Of interest, CTGF can also be expressed in cardiac myocytes under stress,while NFkB blockade reduces cardiac fibrosis and collagen expression [47–50], suggesting that inflammation and hypoxia are likely contributors to cardiac fibrosis. Based on these experimental studies, we can postulate that fibroblasts and their secreted proteins play a role in the transition of the RV from compensated hypertrophy to failure. There appears to be a syncytial relationship between cardiomyocytes, fibroblasts and the cardiac matrix.
In the context of cardiac tissue homeostasis and maintenance of function, a publication by Aranguiz-Urroz et al. is of interest. This study reveals a regulatory connection between β2AR (adrenergic receptors), heart fibroblasts, autophagy, and collagen degradation. Myocardial fibroblasts express more β2AR than β1AR, and stimulation of β2AR in cardiac fibroblasts increased collagen degradation [51]; whether this collagen-degrading consequence of β2AR activation is cAMP dependent is unknown. Finally, we wish to mention that the adipocyte-derived peptide apelin inhibits cardiac fibroblast activation and collagen production [52, 53]. Apelin expression is severely reduced in the failing RV of rats with severe angioproliferative PAH [27].
Endothelial–Mesenchymal Transition
The work of Raghu Kalluri [54–57] points to another mechanism of cardiac fibrosis via endothelial cell–mesenchymal transition (EMT). Although EMT is an embryonic mechanism that is normally dormant in adults, hypoxia, injury, inflammation, or aging can activate this process, and it has been shown that fibroblasts formed in fibrotic lesions in the heart can be of endothelial origin. Type I collagen can induce EMT together with TGFβ, in particular the TGF-β2 isoform [55] and BMP2 and BMP4, while BMP7 inhibits EMT.
Taken together, cardiomyocyte growth, fibroblast activity, and response of cardiac microvessel EC to hypoxia and/or injury engage in a hypertrophy–fibrosis–angiogenesis program that initially allows a compensated response of the RV to pressure overload and stretch. This programmatic response shares many of the characteristics of a wound healing program. The added layer of sympathico-endocrine overstimulation (see below) may tip the balance and throw the switch from compensation to failure.
Dyskinesis, Hypokinesis
Hypokinesis, dyskinesis, failure of the right ventricle to empty during systole, bowing of the interventricular septum, and underfilling of the left ventricle are all described in great detail in previous chapters. These changes are to varying degrees due to regional abnormalities, hypertrophy, fibrosis, or dilatation and may also be related to the different shape of the RV and the underling myoarchitecture and fiber architecture of the RV [19, 20]. Most of the studies that investigated the relationship between cardiac architecture and ventricular function have so far focused on the left ventricle [22, 23] (see also Chap. 17).
Hibernation, Proteolysis, and Autophagy—the Cell Congestive Pathobiology of Right Heart Failure
Cardiac autophagy can be a maladaptive response to chronic hemodynamic stress [58–61] and one hallmark of myocardial hibernation [62–64]. The right ventricle, stressed by a chronically elevated afterload, undergoes hypertrophy which is required for successful adaptation to the chronic stress and strain. As stated, mechanisms which govern the transition from hypertrophy to RV failure are still poorly understood; however, the regulation of protein turnover—synthesis and proteolysis during the transition phase—is critically important. During hypertrophic growth, enhanced protein synthesis leads to an increase in the individual size of the myocardiocytes, whereas decompensation is associated with proteolysis, a switch from cell growth to cell death and replacement fibrosis. Our published data confirm this concept: the hypertrophic RV after chronic pulmonary artery banding is characterized by an increase in the size of the myocardiocytes, a cell growth-directed gene expression pattern and a maintained capillary network [65]. In contrast, in the failing RV the gene expression pattern (RV failure gene expression program) is characterized by a depressed cell growth pattern [27], and tissue analysis reveals increased proteolysis and increased apoptosis. Damaged proteins and organelles, if not eliminated from the cells via autophagy, accumulate and become toxic (proteotoxicity [66]) or trigger apoptotic death [67]. Zhu et al. showed in 2007 that in the setting of left ventricular pressure overload cardiac autophagy is maladaptive and contributes to heart failure progression [58]. The authors also demonstrated increased expression of Beclin 1 levels in pressure-overloaded LV tissues and concluded that Beclin 1 promotes autophagy and LV failure [58]. Importantly and in contrast, autophagy is necessary for the hypertrophic adaptive response of the heart [59]. Because RV hypertrophy is necessary for the successful adaptation of the RV to chronic pressure afterload, inhibition of autophagy during the development of hypertrophy may be detrimental, but inhibition may be beneficial when autophagy has become maladaptive and contributes to heart failure progression. Figure 13.7 is an attempt to construct a mechanistic sequence of events which begins with cardiac stress and has as an end point apoptotic cell death or alternatively a return to cellular homeostasis via organelle and protein clearance, which counterbalances the congestion of the cells with damaged proteins and membrane fragments.


Fig. 13.7
Diagram attempting to construct a mechanistic sequence of events which begins with cardiac stress and has as an end point apoptotic cell death or alternatively a return to cellular homeostasis via organelle and protein clearance, which counterbalances the congestion of the cells with damaged proteins and membrane fragments
Thus, a fundamentally important question is whether in heart diseases autophagy is helpful or harmful. Once the cardiac stress has been initiated, the cells, in order to become not congested or overloaded with toxic proteins, depend on autophagy. Whereas homeostatic during the phase of adaptive hypertrophy, autophagy can become counterproductive leading to the so-called Type II programmed cell death [68, 69]. Whether autophagy is increased in the failing RV of patients with severe PAH or impaired is unknown, but can be presumed. If we can extrapolate from left heart failure (see above), then we would expect maladaptive autophagy in RVF as a component which contributes to RVF progression.
As mentioned, myocardial tissue hibernation is one of the conceptual models of progressive heart failure, which, for a lack of a better explanation, should be used to also explain progressive RVF. Global myocardial ischemia may be a consequence of microvascular dysfunction (see below). Indeed, May et al. generated myocardial hibernation in mice by conditional impairment of VEGF signaling [63]; in this model autophagy activity was increased in association with capillary loss. Once the conditional VEGF inhibition was reversed, hibernation was reversed as the capillary network was restored. These studies are of great interest, specifically because they indicate that microvascular dysfunction, autophagy, and hibernation are potentially reversible. Subsequently this group of investigators applied proteomic analyses and found, in addition to protein expression changes, that hibernation was characterized by a reduced state of phosphorylation of the myosin light chain 2 and of cardiac troponin I, while the abundance of these proteins was unchanged [70]. Unlike myocardial infarction, the hibernating myocardium does not undergo cell death, but can be salvaged; the mechanisms whereby hibernating myocardium survives remain to be elucidated [64]. It is conceivable that mechanisms which control type II programmed cell death play a critical role.
Capillary Rarefaction
Loss of myocardial capillaries is one component of heart microvascular dysfunction. Injury and loss of integrity of remaining capillary endothelial cells is an additional component. Cardiac capillary endothelial cell damage can be demonstrated by electron microscopy (Fig. 13.8) and function defects are reflected in the altered pattern of protein expression that can be shown by immunohistochemistry. As shown and discussed above, VEGF and intact signaling via its receptors is essential for cardiac capillary endothelial cell maintenance [63]. VEGF gene expression is controlled by the transcription factors HIF-1α and PGC-1α. PGC-1α is preferentially expressed in tissues with high energy metabolism; there this transcription factor coordinates genes involved in cellular uptake and mitochondrial metabolism of fatty acids as well as mitochondrial biogenesis. Gomez-Arroyo et al. recently reported a decreased expression in the failing, RV but not in the stressed RV, that responded with adaptive hypertrophy after pulmonary artery banding (PAB) [71]. The altered expression of genes of the PPAR signaling pathways had been first discovered as a result of the microarray gene expression screening analysis of failing RV tissues [27]. Interestingly, HIF-1α expression is not decreased in the failing RV [72], however, the expression of FHL2 (four and a half LIM domains 2) was increased in the failing RV [73], a finding also reported by Mayr et al. [70] in the hibernating myocardium. FHL2 directly interacts with HIF-1α to repress an HIF-1α-dependent transactivation of its target genes. Taken together, one mechanism of capillary rarefaction in the failing RV is a decrease of HIF-1α and PGC-1α-dependent expression of the angiogenic maintenance factor VEGFA. The upstream mechanisms which regulate PGC-1α gene and protein expression in the setting of RVF are still unexplored. We wonder whether the global RV loss of microvessels can be additionally explained by circulating proapoptotic factors such as cytokines or microRNA-containing endothelial cell microparticles [70].


Fig. 13.8
Capillary in the RV-free wall tissue of a rat with experimentally induced RV failure. This electron micrograph shows vesicles and a bleb of the endothelial cells
One important question to address is whether RV hypertrophy per se is sufficient to cause RV ischemia. As will be discussed in Chap. 9, acute occlusion of the right coronary artery, even for a short time, decreases the RV contractile force [74], thus the relationship between RV ischemia and RV contractility is understood and the question whether the hypertrophic RV is ischemic is important. In contrast to the left ventricle, a pressure overload of the RV results in a selective increase in the resting transmural blood flow per gram of hypertrophied RV [75] at least in dogs. Again, in dogs, it has been shown that there is right coronary vasodilation during systemic hypoxia which is to a large extent explained by nitric oxide [76]. Huo et al. [77] analyzed the coronary arterial tree in RV hypertrophy and concluded that RV hypertrophy functionally restores the perfusion at the arteriolar and capillary level. These data agree with older measurements of cross-sectional capillary density and numerical capillary density in RV hypertrophy [78].
Adrenergic Receptor Blockade in Chronic Right Heart Failure
We postulate that apoptosis occurs during the transition from compensated RVH to RV failure and that maladaptive autophagy and capillary loss in the RV contribute to the progression of the RV failure. In this scenario it makes sense to explore therapeutic strategies that would decrease heart cell apoptosis. Beta-adrenergic receptor (BAR) blockers have been used for many years to treat patients with left-sided heart failure and they are highly effective in reducing the morbidity and mortality associated with heart failure [79]. Long-term BAR blockade causes left-ventricular volumes and LVEF to return to normal (reverse remodeling). BAR blockers improve LV remodeling and the mechanisms of this effect may include protecting the myocardiocytes against the toxic effects of catecholamines and reversing the cellular metabolic remodeling [28, 80, 81]. Alternatively BAR blockers, by decreasing heart rate could make the pump work more economical and improve the oxygen supply/demand ratio. Although a number of different BAR blockers have been used, improved outcomes appear to be more frequent when the BAR blocker carvedilol had been used [79], and the authors of the randomized placebo-controlled CHRISTMAS trial tested the hypothesis that carvedilol might improve the function of hibernating myocardium [82]. For more details on carvedilol and RV failure, see Chap. 19.
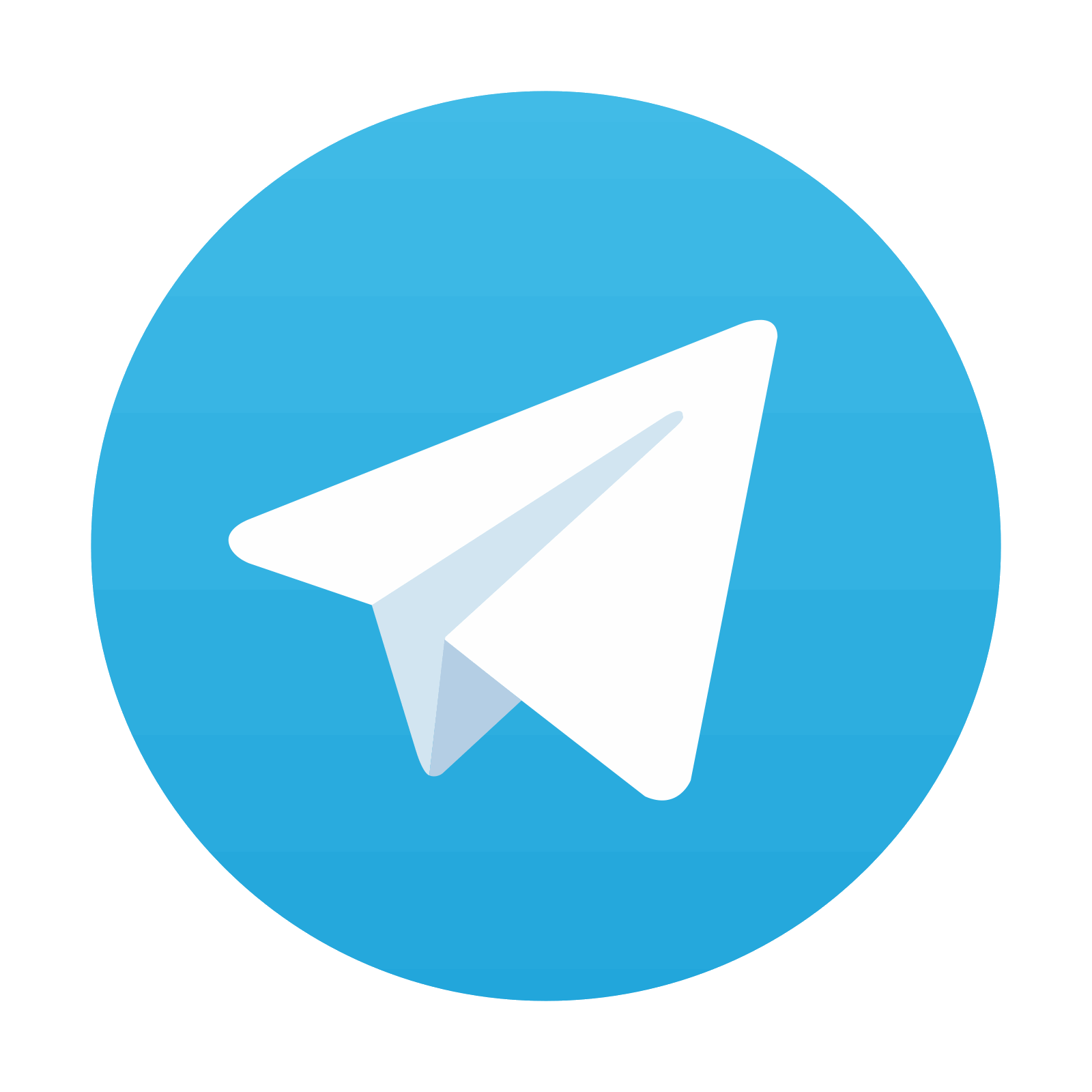
Stay updated, free articles. Join our Telegram channel
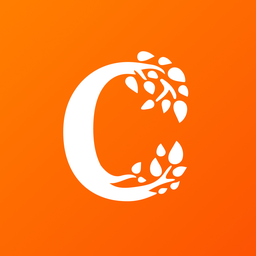
Full access? Get Clinical Tree
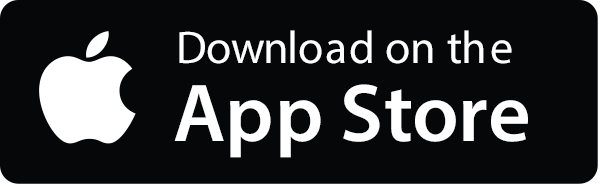
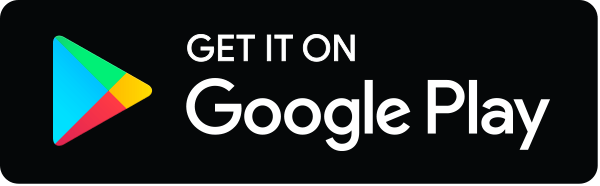