Fig. 3.1
Physiologic changes in the right ventricle during the transition from fetal to neonatal life
In addition to changes in the pulmonary circulation, the fetal myocardium also adapts rapidly during the transition, and the right ventricle (RV) undergoes striking functional and structural changes after birth, summarized in Table 3.1. Due to the presence of “fetal shunts,” communications between the systemic and pulmonary circulations through the patent foramen ovale (FO) and ductus arteriosus (DA) in utero, the left and right ventricles are functionally coupled prior to birth (Fig. 3.2). The RV serves as the “systemic ventricle” in utero, as most of the RV output crosses the widely patent DA and provides 2/3 of combined ventricular output (CVO) in the normal fetus. In fact, RV wall thickness exceeds that of the LV in fetal life, reflecting its dominant functional role in utero. Pulmonary blood flow accounts for only 8–10 % of CVO due to the high PVR and low systemic vascular resistance provided by the placental circulation [1–3]. Left and right atrial pressures and pressures in the great arteries are equivalent due to the widely patent FO and DA, respectively. Whereas pulmonary vasodilation is the central hemodynamic event during the immediate transition, resistance of the systemic circulation rapidly increases due to loss of the low resistance placental circulation and increased systemic vascular tone after clamping of the umbilical cord. Thus, increased systemic vascular resistance, the marked fall in PVR, and functional closure of the “fetal channels” account for the progressive decrease in RV wall thickness and increase in LV mass after birth [7, 8]. Unlike changes in the RV during the normal transition, RV hypertrophy (RVH) persists in the setting of sustained elevations of PVR due to birth at altitude, PPHN, congenital heart disease, and other cardiopulmonary disorders.

Table 3.1
Maturational change in the right ventricle in the fetus, newborn, and infant
Fetus | Neonate | Infant/adult | |
---|---|---|---|
Myocardial structure | Larger non-contractile mass, randomly oriented myofibers | Persistence of increased RV mass | Increased RV chamber size, decreased wall thickness |
Preload | High resting myocardial tension, with decreased compliance | Persistence of relatively poor compliance | Increased compliance, decreased filling pressures |
Afterload | Systemic RV afterload, ejecting into the aorta via the ductus arteriosus | Decreasing afterload following ductal closure and gaseous inflation of the lungs | Continued decrease in afterload over the first 6 weeks of life |
Contractility | Decreased contractility due to structural immaturity and calcium metabolism | High contractility due to increased adrenergic tone | Decreased adrenergic tone, with greater contractile reserve |
Cardiac output | RVCO 1.2–1.5× greater than LVCO | 30 % decrease in RVCO with closure of the PDA and PFO | RVCO = LVCOa |
Substrate utilization | RV free wall perfusion exceeds that of the LV | Decreased RV blood flow and oxygen consumption | Transition to fatty acids as the primary fuel source |
Primary fuel source: carbohydrate |

Fig. 3.2
Prenatal echocardiogram from a normal fetus, illustrating right ventricular ejection of blood into the descending aorta via the ductus arterioles
In addition to these dramatic physiologic changes, the neonatal transition of the RV is further characterized by remarkable cellular, molecular and metabolic adaptations [9]. The fetal heart grows and develops during normal intrauterine life at low oxygen tensions (20–30 Torr) that would induce severe hypoxic stress responses in postnatal life, yet the fetus thrives and is well-prepared for the normal transition at birth. Insights into mechanisms underlying the normal metabolic and functional transition from fetal to neonatal life are not only important for better understanding of neonatal cardiopulmonary diseases, but will also provide insights into adaptive and maladaptive responses in the adult RV. Finally, there is growing support for the concept that perinatal events from fetal life may impact susceptibility for adult disease (“fetal programming”) and the effects of epigenetic signaling on stress responses throughout the “life course” [10, 11].
In this chapter, we discuss mechanisms underlying transition of the RV at birth, including changes in RV metabolism, structure and function in the normal fetus; early changes in the RV during transition at birth and postnatal adaptations; and pathophysiologic features associated with failure of the normal transition associated with PPHN.
Fetal Right Ventricular Metabolism, Structure, and Function
Oxygen Tension, Metabolism, and Substrate Utilization
The normal fetus thrives in its low oxygen environment in utero (PaO2 roughly 18–25 Torr) and this low oxygen tension is essential for normal myocardial growth, development, and functional maturation in utero. The predominant use of carbohydrates for energy substrates, including glucose, lactate, and pyruvate, is a unique feature of fetal myocardial metabolism [12, 13]. ATP generation via these relative oxygen-sparing glycolytic pathways rather than fatty acid oxidation (FAO) represents a key strategy of the fetal heart to tolerate and thrive despite low oxygen levels in normal fetal life [14]. This adaptive feature allows the fetal heart to be more resistant to hypoxia-induced cell injury than the adult heart [15]. Expression of hypoxia-induced genes, such as hypoxia inducible factor (HIF-1) and vascular endothelial growth factor (VEGF) play central roles in modulating myocyte development, myocardial angiogenesis, and fetal heart remodeling [16, 17].
Immediately after birth, however, patterns of substrate utilization switch to FAO. This switch to fatty acid utilization over carbohydrates enhances the efficiency of myocardial ATP production, a pattern that is largely sustained throughout adult life [18]. However, myocardial metabolism remains capable of late adaptations that allow switches in substrate utilization. Mechanisms generally involve the “Randle Cycle,” in which FAO attenuates glucose oxidation via feedback inhibition to adapt myocardial metabolism to different forms of nutrient supply with injury or stress [19–21].
This normal metabolic switch at birth accompanies the expression of “adult” isoforms of metabolic enzymes and other proteins. However, in diverse conditions, such as hypoxia, ischemia, hypertrophy, atrophy, diabetes, and hypothyroidism, the postnatal heart may revert to the “fetal” gene program [14, 21]. These adaptive mechanisms are also a feature of failing heart muscle, where at a certain point, this fetal-like reprogramming no longer suffices to support cardiac structure and function. Metabolic regulation in the postnatal heart likely plays a critical role mediating gene expression in response to stress, which potentially protects the stressed myocardium from severe functional impairment and apoptosis. That is, with stress due to ischemia, pressure or volume overload, the RV adjusts metabolic function to switch its preferential substrate from fatty acids to carbohydrates. As glucose metabolism requires less oxygen consumption for an equivalent amount of ATP generation, this is likely a successful adaptive strategy. However, long-term dependence on glucose utilization for ATP generation is not efficient and can lead to energy starvation and cardiac failure [22]. These events may be mediated by long-term changes in regulation of critical “fetal genes,” such as protein kinase C epsilon, heat shock protein 70, and endothelial nitric oxide synthase (eNOS), which can predispose the developing heart to increased susceptibility to injury and induce myocardial dysfunction [21]. With aging, physiologic suppression of glucose oxidation by fatty acids is altered, with greater dependence on glucose metabolism and relatively less FA use [23]. Importantly, decreased RV fatty acid uptake and oxidation has been demonstrated by PET studies in humans with pulmonary hypertension [24].
The partial pressure of oxygen in the blood entering the RV is significantly lower than the blood entering the LV, by virtue of the fact the oxygen-rich blood returning from the placenta is directed through the widely patent FO by the Eustachian valve. This distribution of fetal blood flow maximizes oxygen delivery to the brain and coronary circulations in utero and protects against hypoxic injury [25]. Myocardial oxygen consumption studies showed no difference between fetal and adult sheep [13], suggesting that the fetal RV has relatively higher myocardial blood flow. Other experiments in which myocardial blood flow was measured in fetal lambs showed greater oxygen delivery to the RV free wall, as compared to the LV free wall [26]. The right side of the ventricular septum receives more coronary flow than the left side in utero, which is reversed in the adult [26]. This pattern reflects the RV’s role as the dominant functional ventricle in utero, with higher oxygen utilization.
Although physiologically normal, the low oxygen tensions in the healthy fetus are necessary for normal cardiac development, yet a further drop in oxygen tension due to intrauterine stress readily becomes pathologic and mediates harmful effects that cause myocardial injury and severe dysfunction during the neonatal transition as in PPHN. Perinatal hypoxia causes marked LV and RV dysfunction with sustained pulmonary hypertension after birth, which can masquerade as “primary” pulmonary vascular disease [5].
In addition, prenatal events can alter gene expression patterns in fetal hearts and related signaling patterns that may increase susceptibility for adult disease, known as “Barker’s Hypothesis.” [27]. For example, myocardial growth, function, and gene regulation may be persistently altered by prolonged intrauterine hypoxia, hemodynamic stress, inflammation or nutritional deficits. This concept of persistent disease after disruptions of normal fetal programming, largely through epigenetic mechanisms, has recently been supported in a study of young adults who were prematurely born [28]. In this study, RV structure and function were quantified by magnetic resonance imaging in preterm-born young adults and compared with term controls. RV ventricular end diastolic volume was significantly smaller and RV mass increased in former preterm adults [28]. In addition to changes in myocardial structure, RV ejection fraction (RVEF) and systolic function remained lower in the preterm population, demonstrating a persistent impact on the RV, long after the perinatal events related to premature birth. These findings were more striking than was previously observed in assessments of LV structure and performance in these patient groups [29].
Fetal RV Structure
The fetal myocardium differs from that of the postnatal heart in several important ways, shown in Table 3.1. Electron micrographs (Fig. 3.3) of the fetal myocardium show smaller myocytes and a greater proportion of “non-contractile mass,” consisting of nuclei, mitochondria, and cell membranes [12]. In fact, only 30 % of the fetal myocardium contains contractile mass in contrast to 60 % in the adult [12]. The diameter of fetal cardiomyocytes is only 5–7 μm, whereas adult myocytes are much larger, roughly 15–25 μm [30]. The increase in muscle mass during gestation is almost exclusively the result of an increase in cell numbers [30]. Fetal myocardial nuclei are large and polyploidy is unusual. In the early gestation fetus, the longitudinal orientation of the sarcomeres is more random, but the fibers become more parallel as the fetus nears term [1–3, 12]. Evidence from studies of human fetuses suggests that the LV and RV grow at the same rate [2, 31]. The fetal heart grows by rapid cardiomyocyte proliferation early on in gestation, but then loses the ability to propagate with further myocyte differentiation during late gestation. The fetal heart responds to stress with hypertrophy of existing cardiac cells, unlike the adult cardiomyocyte, which is in a more differentiated state and has less ability to divide.


Fig. 3.3
Electron micrographs illustrating change in myocardial structure illustrating smaller myocytes with larger non-contractile mass of the fetus (upper panel) in comparison with the postnatal myocardium (lower panel). (From Smolich et al. [46])
Fetal RV Function
Preload
With regard to preload, the fetal heart operates at the upper limit of the Starling curve (Fig. 3.4). Studies in fetal lambs have shown that a 10 % decrease in blood volume decreases CO, whereas a 10 % increase in volume elevates atrial pressures without changing the CO [4–6, 32, 33]. Data from animal studies have clearly demonstrated that the fetal RV has a higher resting tension than the adult RV, indicating lower ventricular compliance in utero [1–3, 12, 34]. This inability to tolerate additional preload, where even small increases in intravascular volume may dramatically increase central venous pressure, may lead to congestive heart failure and hydrops fetalis; this is characterized by pleural effusions, anasarca, and high perinatal mortality. Interventricular interactions between the fetal RV and LV are also particularly striking, as even small changes in LV pressure reduce RV preload [7, 8, 12].


Fig. 3.4
Differences in the relationship between filling pressure and stroke volume in the fetal and adult right ventricles. As shown, stroke volume is markedly increased for a given right atrial pressure in the adult when compared with the fetal RV. (Adapted from Rychik J et al., [35])
Afterload
In utero, the RV ejects blood into the pulmonary trunk, which trifurcates into the left and right pulmonary arteries and the ductus arteriosus (DA) (Fig. 3.5). Most of the RV output crosses the ductus arteriosus due to high PVR, and the RV serves both the pulmonary and systemic circulations. The aortic isthmus, the section of the aorta between the left subclavian artery and the insertion of the DA, is narrower than the descending aorta, which functionally separates the two circulations to a small extent [8, 9]. RV and LV wall thickness are approximately equivalent in utero, resulting in a greater wall thickness to radius ratio for the RV. According to La Place’s law, this results in greater RV wall stress [10, 11, 35], and causes greater sensitivity to changes in afterload [12, 13, 36].


Fig. 3.5
Schematic illustrating perinatal changes in pulmonary vascular resistance and blood flow in normal and PPHN infants. (Adapted from Rudolph AM, 2004)
Due to the widely patent DA, the fetal RV is more affected by increased systemic vascular resistance than high PVR. Acute elevations of fetal systemic blood pressure reduce RV stroke volume [16, 17, 37]. Sustained elevation of systemic arterial pressure causes striking RVH, pulmonary hypertensive vascular disease, and RV failure in severe cases. Thus, increased fetal PVR increases the risk for PPHN at birth, but is not sufficient in itself to cause RVH or RV failure in utero, without associated hemodynamic stress (e.g., closure of the DA or systemic hypertension [18, 38, 39]. Banding of the pulmonary artery (see also Chaps. 5 and 22) in fetal sheep to simulate pulmonary stenosis causes striking RVH. Over time, some fetuses develop a large, dilated tricuspid valve and RV, whereas others develop a hypoplastic right AV valve and ventricle [19–21, 30]. Factors determining which pathway (RVH or dilation with an underdeveloped RV) is followed remain uncertain.
Contractility and Cardiac Output
Contractility of the fetal heart is less than that of the adult myocardium at similar muscle lengths [12, 14, 21]. Isometric force development, including the extent and velocity of shortening at any load, are reduced in the fetus when compared to the adult [12, 22]. This is partly due to structural immaturity of the myocardium, from fewer sarcomeres and the lack of parallel orientation of myofibrils. Calcium management is impaired in fetal cardiomyocytes due to maturational differences in the t-tubule system of the sarcoplasmic reticulum (SR) [21, 40]. Isolated fetal vesicles with SR have a 60 % decrease in active accumulation of calcium, lower calcium pump protein expression, and activity [23, 40]. Decreased sympathetic innervation of the fetal heart and variability in adrenergic receptors also reduce fetal myocardial contractility [8, 24]. Human RV output in utero is 1.2–1.5 times that of the LV, representing 60–70 % of CVO [25, 35]. At 20 weeks gestation, ovine pulmonary blood flow is around 13 % of the CVO, which increases to 20 % by 30 weeks [13, 41]. Cardiac MRI has recently been used to quantify pulmonary blood flow and oxygen saturation in human fetuses, and these results have been consistent with past studies [26, 42]. Flow patterns in the branch pulmonary arteries are very characteristic in the fetus, consisting of a short period of forward flow (first third of systole), followed by backflow, which extends throughout the remainder of systole into diastole [8, 26]. This deflection, known as the peak early diastolic reversed flow, is due to reflection of the pressure wave generated by the RV when it encounters high resistance in the pulmonary vascular bed [5, 43] (see also Chap. 4).
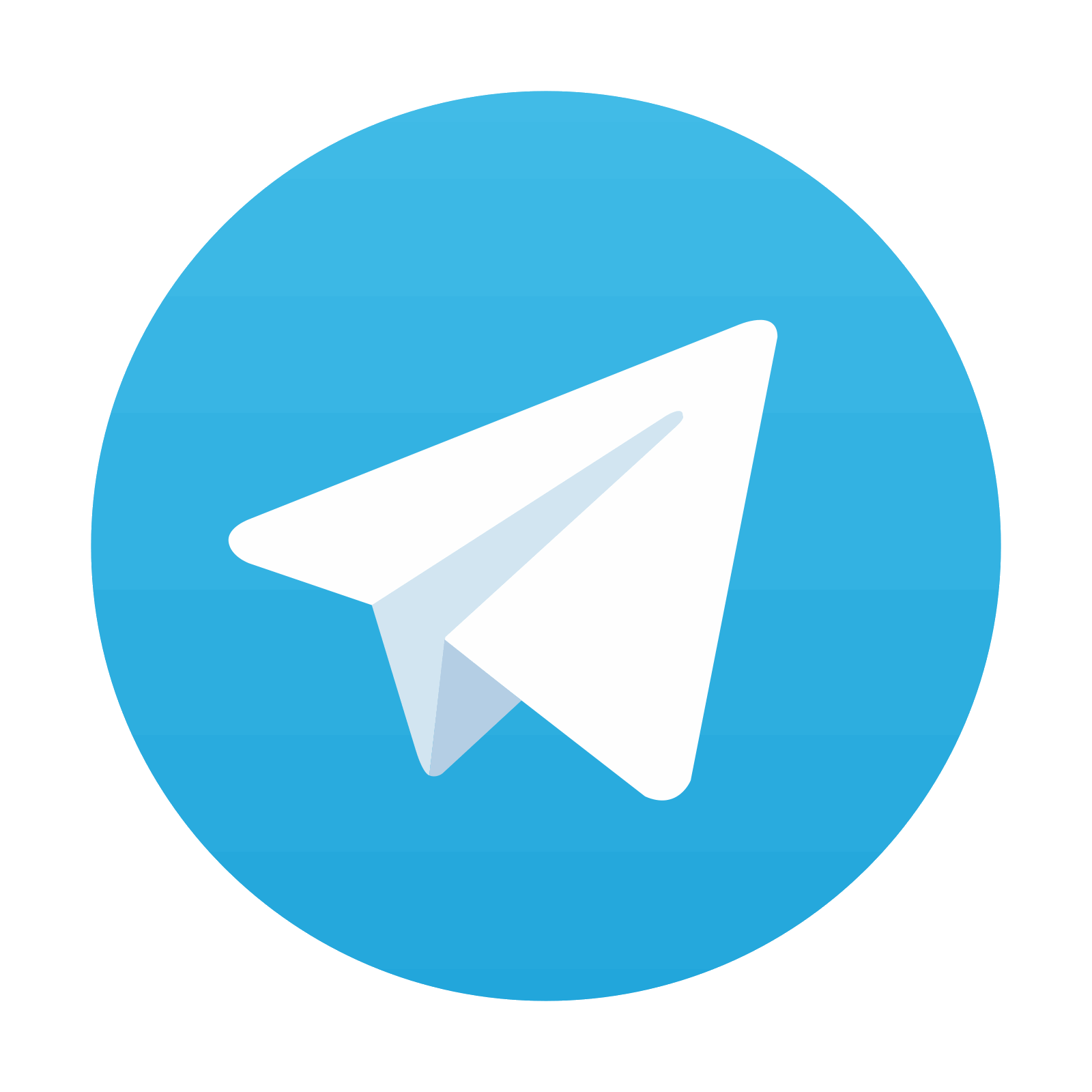
Stay updated, free articles. Join our Telegram channel
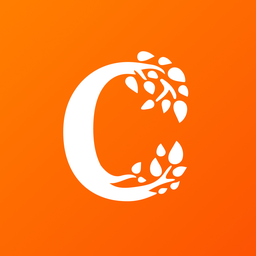
Full access? Get Clinical Tree
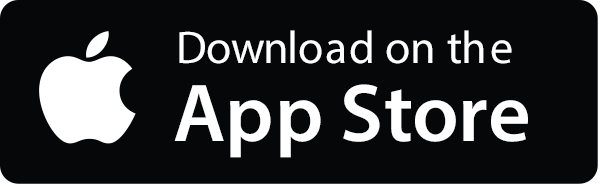
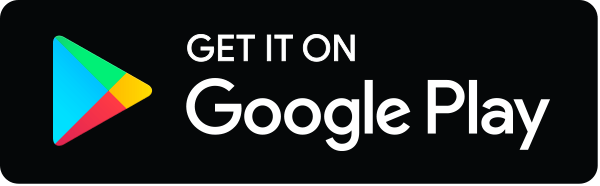