Fig. 8.1
Schematic of key microvessels and their organisation. On the arterial side is the arteriole and metarteriole. The metarteriole connect directly with the venule (on the venous side) forming a route (labelled as the ‘preferential channel’) which bypasses the capillary bed. Capillaries lie between the metarteriole and the venule; the capillary bed resembles a mesh rather than a bifurcating network. Precapillary sphincters are bands of smooth muscle which control flow in the capillary bed
Arterioles (diameter 10–100 μm). These vessels are on the arterial side of the circulation. These branch several times and link the small muscular arteries to the capillaries. Arterioles contain three layers (intima, media adventitia), of which the media is proportionally the largest. The media is primarily comprised of vascular smooth muscle cells (typically, one or two layers in the larger arterioles with only a single spiralling layer in the smaller vessels leading to the capillaries). The residual tension in these cells, their tone, allows for alteration of arteriolar diameter and thus lumen size in response to changes in neural stimuli or in local chemistry. This plays a large part in control of capillary bed flow (see below) as well as the control of vascular resistance and systemic blood pressure.
Metarteriole (diameter 10–20 μm). This term refers to an arteriole which is directly connected to a venule. This provides a vascular shunt which allows the capillary bed to be bypassed. In terms of structure, whilst metarterioles do not have continuous media, smooth muscle cells are present at the distal end of these vessels at the entrance to the capillary bed forming what are known as ‘precapillary sphincters’. These can constrict to reduce flow into the specific capillary bed.
Precapillary sphincter. The precapillary sphincter is a band of smooth muscle cells which controls flow into the capillary.
Preferential channel. This is the channel between the metarteriole and the connecting venule. Constriction of the precapillary sphincters results in most flow travelling through the preferential channel rather than through the capillary bed.
Capillaries (diameter 4–10 μm; up to 40 μm in sinusoidal capillaries). These are the smallest vessels in the cardiovascular system and, structurally are characterised as three types; continuous, fenestrated and sinusoidal. Capillaries consist of an endothelial layer and a basement membrane. Continuous capillaries have an uninterrupted endothelial lining and are the most common type of capillary. Fenestrated capillaries have pores in the endothelium which allow passage of certain molecules and are commonly found in endocrine glands, the gastrointestinal tract and the glomeruli of kidneys. Sinusoidal capillaries have large gaps in the membrane and an incomplete endothelial coverage which allows the flow of fluid and large molecules into the interstitial space. Sinusoidal cells are mostly found in the liver.
Venule (10–200 μm). These vessels are on the venous side of the circulation, branching several times between the capillaries and the veins. Venules contain three layers (intima, media, adventitia), but these layers are much thinner than for arterioles and the medial layer is almost absent.
The above microcirculation architecture is commonly taught in textbooks. However, it has been pointed out that it is only the GI tract which has metarterioles in the sense of an arteriole with a discontinuous medial layer and precapillary sphincters. In other circulatory beds the term ‘metarteriole’ has come to refer to the smallest arterioles immediately before the capillaries.
It can be seen both from Fig. 8.1 and the discussion above that the capillary bed is not a conventional bifurcating network. There are bypasses in the form of ‘preferential channels’; if precapillary sphincters are activated this can result in almost complete shut down of flow to the capillary bed. The capillary bed itself more resembles a mesh than a bifurcating network, so that there are multiple possible routes that a red cell can take through the bed.
8.1.2 Functions
The main functions of the microcirculation in terms of its components parts can be summarised as follows:
Resistance to flow. Constriction of smooth muscle in the arterioles and the metarteriole enables control of local vascular resistance. This enables control of the pressure at the level of the capillaries and flow rate through the capillary bed.
Molecular exchange. The exchange of fluid and of key molecules occurs through the walls of the capillaries. The main mechanism of exchange (diffusion) is pressure driven hence it is important to maintain hydrostatic pressure constant at the level of the capillary.
Flow bypass. This refers to blood flow in vascular beds where the microcirculation contains a preferential channel controlled by precapillary sphincters. This allows particular capillary beds to be excluded from the microcirculation.
Capacitance. The venules act as a reservoir of blood; some 22 % of the whole blood volume is contained in the venules.
8.2 Haemodynamics and Mechanics
8.2.1 Pressure and Velocity
In Chap. 2, basic concepts of cardiovascular biomechanics were introduced where it was noted that both blood pressure falls and blood velocity falls while travelling from the aorta to the capillaries. Rough estimates were provided in Table 2.1 of mean velocity based on a pure bifurcating model. Data on pressure and velocity in the microcirculation is shown in Fig. 8.2. This shows considerable decrease in pressure in the arteriolar part of the microcirculation, which is due to the high resistance to flow of arterioles. The pressure continues to fall through the capillary bed and the venules. Blood velocity is lowest in the capillaries with a value of around 2 mm s−1. Table 8.1 shows values of mean velocity and other haemodynamic quantities in different vessels of the microcirculation.


Fig. 8.2
Blood pressure and velocity in the microcirculation from arterioles to venules. From Zweifach BW, Lipowsky HH; Quantitative studies of microcirculatory structure and function. III. Microvascular hemodynamics of cat mesentery and rabbit omentum; Circulation Research; Vol. 41(3), pp. 380–390. Copyright American Heart Association (1977), reprinted with permission from Wolters Kluwer Health, Inc.
Table 8.1
Haemodynamic quantities in the microcirculation
Vessel | Diameter (μm) | Mean velocity (mm s−1) | Reynolds number, Re | Womersley parameter, α | Pseudoshear rate (s−1) | Wall shear stress (Pa) | Effective viscosity (mPa s) | Haematocrit |
---|---|---|---|---|---|---|---|---|
Arterioles | 60 | 12 | 0.2 | 0.8 | 200 | 6 | 2.8 | 0.29 ± 0.12 |
Arterioles | 15 | 7 | 0.03 | 0.2 | 470 | 14 | 4.9 | |
Capillaries | 5 | 0.2 | 0.0003 | 0.07 | 40 | 12 | 15 | 0.23 ± 0.14 |
Venules | 18 | 0.2 | 0.001 | 0.2 | 10 | 3 | 4.2 | 0.31 ± 0.13 |
Venules | 72 | 2.4 | 0.05 | 0.9 | 30 | 10 | 2.9 |
8.2.2 Blood Flow
Blood is a suspension of particles, principally red cells. The human red cell dimensions of 7.5 by 2 μm, is tiny in comparison to the diameter of 1–30 mm of the larger arteries and veins. This means that in larger arteries and veins the blood can primarily be considered as a continuous fluid; or in other words, individual red cells can usually be ignored in considering haemodynamics. However in the microcirculation, the dimensions of the vessels are close to the dimensions of the red cell. This is especially true for capillaries where the typical diameter of 4–10 μm is comparable to the 7.5 μm diameter of the red cell. Red cells squeeze through capillaries in single file and are distorted in shape as they do so. Flow in the microcirculation is more complex than flow in larger vessels, and the effect of individual red cells must be considered. Chapter 3 describes a number of phenomena concerning flow of particles in tubes where the ratio of tube-diameter to particle-diameter is low and/or where shear rate is low. These phenomena are relevant to the microcirculation and are briefly summarised here.
Cell margination and depletion. Particles flowing in a cylindrically shaped vessel will experience a number of forces. Some forces will push an individual particle away from the centre of the vessel and others will push the particle away from the vessel wall. This can lead to accumulation of particles in a ring between the vessel centre and the vessel wall. This is known as the Segre–Silberberg effect from the original paper (Segre and Silberberg 1962). Flow in blood is more complicated in that there are several different particle types; red cells are relatively deformable while platelets and white cells are relatively stiff. There is interaction between the particles in flow of whole blood. The red cells have a tendency to move away from the wall, leaving a layer near the wall depleted of red cells, while platelets and white cells are pushed by the red cells to the vessel wall (Aarts et al. 1988) as shown in Fig. 8.3.
Fig. 8.3
Simulation of flow or red blood cells (RBCs) and platelets in a vessel with a diameter of 20 μm. Red cells are aligned with the direction of flow and there is a cell-free region near the wall. Platelets in yellow appear mainly in the cell-free region (having been pushed there by the RBCs). From; Rheologica Acta, Effect of tube-diameter and capillary number on platelet margination and near-wall dynamics, 2015, doi:10.1007/s00397-015-0891-6; Krüger T; © Springer-Verlag Berlin Heidelberg 2015, with permission of Springer
Variation of viscosity with vessel diameter. Due to red cell depletion near the wall, the effective viscosity of the fluid is reduced when the vessel diameter is a few red blood cell diameters. Near the wall, the particle density is reduced and the viscosity is dominated by the underlying fluid. The viscosity mainly arises from flow in the high shear region near the wall, so that the overall viscosity is more akin to that of the fluid base rather than that of the suspension. This is called the Fahraeus–Linqvist effect after the authors of the original paper (Fahraeus and Lindqvist 1931). Calculations of the Fahraeus–Lindqvist effect shown in Fig. 3.15b demonstrate a minimum viscosity at a vessel diameter of around 7–9 μm depending on haematocrit. In smaller vessels the red blood cell is deformed and hence viscosity is higher.
Variation of haematocrit with vessel diameter. This is concerned with reduction in haematocrit within the vessel compared to that within the receiving reservoir. This is called the Fahraeus effect after the author of the original paper (Fahraeus 1929). The explanation is similar for the Fahraeus–Lindqvist effect in that the red cells in the centre of the vessel move faster than the liquid at the edge of the vessel. Hence the relative volume of red cells to plasma is greater in the discharge fluid than for the fluid in the tube. Figure 3.15a shows that the effect is greatest (i.e. largest reduction in haematocrit) for a vessel diameter of 12–13 μm.
Red cell aggregation. At low shear, red cells aggregate leading to an increase in viscosity and further exacerbating red cell depletion at the wall.
Building on the above understanding we will now look at a number of features of flow in the microcirculation. Further details of flow in the microcirculation are provided in review articles, e.g. Pries et al. (1996), Mchedlishvili and Maeda (2001), Baskurt and Meiselman (2003).
Plasma skimming. This is the phenomenon whereby flow in a side-branch contains few or no red cells as a result of cell depletion at the wall of the parent vessel (Fig. 8.4). Through the microcirculation and in the capillary bed the red cell density will be highly variable depending on a number of factors including the diameter of the vessel, red cell aggregation and the flow rate.
Fig. 8.4
Illustration of the flow of red cells in the microcirculation. There is a tendency to axially accumulate in the main vessel leading to a cell-free region near the wall. In one side-branch this leads to plasma skimming. In another branch red cells travel in single file
Viscosity variations. The effective viscosity is dependent on the red cell density, the diameter of the vessel, red cell aggregation, the flow rate and the exact path which is taken by the red cells through the microcirculation. These factors lead to considerable variation in viscosity in vessels of similar diameter, and variations in viscosity with diameter. Table 8.1 shows the highest viscosity of 15 mPa s for capillaries (compared to 3.5–4 mPa s in large arteries), and low viscosities of 2.8–2.9 mPa s in the large arterioles and venules.Stay updated, free articles. Join our Telegram channel
Full access? Get Clinical Tree
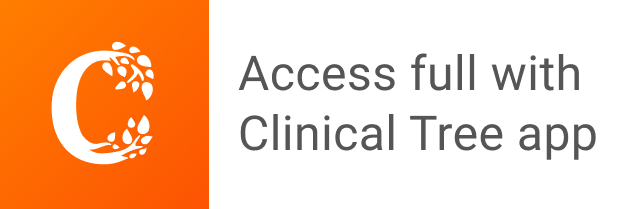