Educational Aims
The reader will gain an improved understanding of:
- •
Key terminology used in microbiome research for respiratory paediatrician.
- •
The methods used to investigate microbial communities in the lungs, including their advantages and limitations.
- •
Differences in the development of lung microbiota in preterm and term infants.
- •
Associations between lung microbiota in chronic lung disease in children and the contribution role of oral taxa in disease.
- •
The gut-lung axis.
Abstract
The advent of next generation sequencing has rapidly challenged the paediatric respiratory physician’s understanding of lung microbiology and the role of the lung microbiome in host health and disease. In particular, the role of “microbial key players” in paediatric respiratory disease is yet to be fully explained. Accurate profiling of the lung microbiome in children is challenging since the ability to obtain lower airway samples coupled with processing “low-biomass specimens” are both technically difficult. Many studies provide conflicting results. Early microbiota-host relationships may be predictive of the development of chronic respiratory disease but attempts to correlate lower airway microbiota in premature infants and risk of developing bronchopulmonary dysplasia (BPD) have produced mixed results. There are differences in lung microbiota in asthma and cystic fibrosis (CF). The increased abundance of oral taxa in the lungs may (or may not) promote disease processes in asthma and CF. In CF, correlation between microbiota diversity and respiratory decline is commonly observed. When one considers other pathogens beyond the bacterial kingdom, the contribution and interplay of fungi and viruses within the lung microbiome further increase complexity. Similarly, the interaction between microbial communities in different body sites, such as the gut-lung axis, and the influence of environmental factors, including diet, make the co-existence of host and microbes ever more complicated. Future, multi-omics approaches may help uncover novel microbiome-based biomarkers and therapeutic targets in respiratory disease and explain how we can live in harmony with our microbial companions.
Nomenclature
Term
Definition
Microbiota
The interacting bacteria, archaea and fungi .
Microbiome
The microbiota, their functions and viral elements .
Lungs/Lower Airway Microbiota
Microbiota sampled from anatomical sites below the vocal cords, outlined in Fig. 1 . The tracheal aspirate (TA), sputum or bronchoalveolar (BALF) microbiota refers to the microbes collected from the lower airways via the respective sampling methods.
α-diversity
Diversity within an individual/sample. Characterised by species richness (number of different taxa) and evenness (the relative abundance of different taxa), or both .
β-diversity
Comparison of microbial communities between individuals/samples. Generally based on distances between points on a multidimensional plot .
Introduction
When one considers the importance of communities in biological systems and ecology, it is important to reflect that human society thrives on diversity and dynamism – the same could be hypothesised for the lung microbiota.
The Human Microbiome Project (HMP), undertaken in 2007, marked a turning point in microbiology with a rapid shift away from the reliance on culture-based techniques towards molecular/genetic characterisation. HMP set out to characterise the microbial colonisers at various mucosal surfaces in humans answering the question “what microbes are there”, whilst also providing links between microbiota in health and disease . Yet, the lungs were not explored, likely due to the long-held belief that the lower airways were sterile . Hilty and colleague’s seminal work produced a paradigm shift, revealing an array of bacterial genetic material which differed between the healthy/diseased lungs of children and adults – the “lung microbiota” . Such findings stimulated interest in the role of lung microbiota and the application of ecology theory to gain a better understanding of chronic respiratory diseases .
The lung microbiota
The respiratory tract can be anatomically classified into upper and lower compartments, with the lower airways conferring their own unique microbiota, is the least understood microbiota niche within the total “airway system” ( Fig. 1 ) and may be critical to pulmonary development in early life.

“The Hygiene Hypothesis” suggests that more diverse microbiota-host interactions are beneficial to host health . Furthermore, In vivo evidence supports causal relationships between lung microbiota and lung physiology . In murine neonates, the absence of airway commensals disrupts pulmonary tolerance to allergen exposure, by inhibiting the formation of regulatory immune cells, and is linked to development of experimental asthma. This process can be abrogated by introducing lung commensals in the first 2 weeks of life, but not afterward, suggesting a ‘window of opportunity’ exists shortly after birth where microbiota-host interactions shape pulmonary development . Lung microbiota differences have been demonstrated between term and preterm infants in the first weeks of life. Clinically, the preterm lung microbiota is less diverse than full term counterparts, suggesting lung microbiota could in part explain the observation that premature infants are at higher risk for the development of chronic respiratory issues ,
Furthermore, unlike the gut and oral microbiota which are thought to be relatively stable, the lungs likely possess a ‘dynamic’ polymicrobial environment which exhibit bidirectional movement (upward and downward) within the airways . Lung microbiota are predominantly derived from the oral cavity/ oropharynx although the relative abundances of microbiota species differ along the respiratory tract . Microbiota translocate into the lungs mainly via micro-aspiration of bacteria and inhalation of fungal spores . Microbial load declines distally in the lower airways with numbers 100-fold lower at alveolar regions than at the oropharynx [ Fig. 2 ]. Immigration events are finely balanced by clearance mechanisms in the lungs. Immune factors neutralise foreign antigens whilst the mucociliary system sweeps foreign particles upwards and out of the lower airways. Additionally, the lungs naturally possess an array of “local factors”, creating a harsh environment that inhibit microbial growth . This microbial immigration-to-clearance equilibrium maintains a dynamic, diverse, but low abundant, microbiota – potentially a marker of lung health .

In chronic lung disease, disruption to local factors can create an imbalance between microbial immigration and clearance mechanisms, leading to disrupted microbial communities in the lungs which may contribute to disease processes . This transition from dynamism to stability may be a key metric of severe respiratory decline . Moreover, the increased presence of oral taxa in the lower airway may contribute to inflammatory processes and worse outcomes . For example, in CF, impaired lower airway clearance mechanisms can create niche opportunities that permit an increase in the abundance of oral taxa, in turn, providing nutritional support for pathogenic growth in the lungs . Furthermore, increased presence of orally derived microbiota within the lower airways of children with asthma have been linked to exacerbations .
Herein, we examine clinical evidence comparing lung microbiota composition between healthy term and preterm infants and review microbiota involvement in three chronic lung conditions: BPD, asthma and CF. Additionally, we consider whether oral-associated taxa may promote disease processes in asthma and CF.
Although general microbiological trends emerge, high variability is commonly reported between patients, indicating highly personalised lung microbiota, like data from gut studies . Relationships between lung microbiota diversity and paediatric lung disease is unclear with the exception of more advanced stages in CF, suggesting taxonomic descriptions alone may be insufficient, underlining the need to combine microbiota presence together with functional outputs such as the metabolites generated and genes expressed in samples which is currently lacking . The issue of obtaining appropriate lower airway samples in paediatrics and handling specimens that have low microbial biomass have complicated research. Further challenges reflect technical issues with experimental reproducibility, and availability of appropriate controls .
Despite these challenges and complexities, it is essential that respiratory paediatricians understand the role of microbiota in lung disease since future treatment strategies may involve manipulating lung microbiota to improve disease outcomes .
Methods in lung microbiome research
Historically, our understanding of pulmonary microbiology has been driven by a reliance on culture-based microbiology and the need to “grow” bacteria on growth media in particular environmental conditions. The arrival of amplicon sequencing ( Fig. 3 ), mediated by next-generation sequencing (NGS) technologies, and metagenomics, overcomes these culture-dependent biases. DNA from hard-to-culture anaerobic organisms has been consistently identified in the lungs. These approaches are rapidly challenging our understanding of lung microbiology, however, NGS also confer limitations.

Sequencing the lower airways – what is there?
Amplicon sequencing
Bacteria are the main contributors to the lung microbiome . Molecular probes target hypervariable portions (V1-V9) of the bacterial/archaeal 16S rRNA gene [ Fig. 3 ]. Selectively targeting a particular hypervariable region can identify bacteria/archaea down to the genus level, however, the choice of hypervariable region amplified can significantly impact downstream results, since no single region can discriminate between all taxa . Full-length 16S sequencing may provide species-level resolution but has rarely been applied to date . Prevotella , Veillonella and Streptococcus may account for approximately one-third of species in healthy lungs . Others have found that, in some individuals, the heathy lung microbiota signatures are indistinguishable from microbial DNA found in negative control samples, whilst the identification of orally-derived taxa, such as Prevotella and Veillonella, are associated with pro-inflammatory responses and confer a higher microbial load in asymptomatic subjects .
Fungi and viruses are less well characterised in the airways. Fungi can be examined by targeting the 18S rRNA gene or internal transcriber spacer (ITS)-1 or ITS-2 regions and are found at low levels in health with prevalent taxa including Aspergillus and Cladosporium . The durable fungal cell wall requires a more vigorous cell-lysis approach to release DNA prior to nucleic acid extraction, though this can also be relevant to a lesser extent for Gram-positive bacteria . Viruses do not contain highly conserved genetic elements, however, can be investigated via metagenomic sequencing .
Shotgun metagenomics
Shotgun metagenomics sequences all DNA within a sample, providing the opportunity to analyse the entire microbiome including DNA-based viruses and the functional capacity of microbes, for example the presence of antimicrobial resistant genes in bacteria .
Bacteriophages may be the predominant viral entity in the lung; however, further work is required to fully elucidate the role of all “commensal” viruses, including RNA-based viruses, within the lungs .
Technical limitations have impacted the use of metagenomics in lung microbiome studies . The high host-to-microbe genetic content in samples means microbial enrichment (especially viral enrichment) and/or host depletion methods are necessary. Low viral-host genetic material ratio in the lungs means accurate viral analysis requires different sample processing compared to samples interrogated for bacterial/fungal DNA .
Similarly, because microbial sequences identified need to be matched against databases of known organisms, fungal and viral sequences are poorly represented compared to bacteria .
Limitations of DNA-based sequencing approaches
Since DNA-based sequencing approaches are unable to differentiate between live and dead microbes, methods have been developed that can deplete so-called relic DNA . Yet, relic DNA may still induce physiological effects via interactions with host immune factors and therefore contribute to the disease process .
Thus, studies are needed that complement DNA-sequence approaches with functional investigations, providing a more comprehensive understanding of microbiota-host relationships. Metatranscriptomics can provide information on gene expression of host and microorganisms and elucidate RNA-based viruses . Metabolites and proteins present in specimens can be investigated via metabolomics and proteomics, respectively. These approaches can be used to correlate microbiota changes to functional profiles within lower airways to determine possible “cause and effect” between the lung microbiome and pulmonary health/disease, particularly in longitudinal studies . Such approaches may establish novel “endotypes” – subclassifications of disease based on microbiome markers, as has been attempted in paediatric asthma . Multi-omics strategies may also help explain the high variability commonly observed between individuals in lung microbiota studies .
Sampling the paediatric lung
Lung microbiota specimens in children are mainly collected during flexible bronchoscopy, sputum (spontaneously produced or induced) or tracheal aspiration (TA). All sampling modalities confer strengths and limitations and reflect distinct microbial niches within the lower airways, making cross-comparisons challenging .
Bronchoscopy
Bronchoscopy sampling via bronchoalveolar lavage fluid (BALF) can collect from 1/40 of total lung surface area including alveolar regions, whilst the use of a specimen brush may manage to dislodge mucosal-adherent taxa in the conducting airways. However, paediatric bronchoscopy is performed under general anaesthesia and are reserved for children with respiratory complications, making serial sampling and the recruitment of healthy children difficult .
Sputum
Sputum collection is arguably the most readily available and feasible option for serial sampling of the lungs, although younger children cannot expectorate and those who can typically present with more severe disease . Induced sputum (via inhalation of nebulised saline) may permit inclusion of healthy children in studies, although may be deemed unethical in infants, restricting the recruitment of younger cohorts . Furthermore, sputum must traverse the upper airways increasing risk of contamination from the more abundant upper airway microbiota .
Tracheal aspirate
Children that are intubated during a procedure or receiving mechanical ventilation for other reasons can permit TA sampling from the endotracheal tube, with the latter allowing serial sample collections. TA microbiota is likely distinct from samples collected more distally and may represent a mixture of microbiota entering from the upper airways and microbiota being removed from the lungs . Samples collected two-hours post intubation have also been shown to underrepresent obligate anaerobes and/or overrepresent aerobes compared to TA samples taken at time of intubation . Therefore, it may be to good practice to report the duration of intubation prior to sample collections in studies.
Although there is no established gold-standard method of sampling, protected bronchoscopy may be the most effective to minimise pharyngeal contamination at time of sampling, since contamination is a major consideration in lower airway microbiota studies .
Low-biomass contamination
Lower airway specimens contain a low microbial load or “low-biomass”. Low-biomass samples follow a “power-law dynamic”, meaning the lower the biomass the greater the impact of sample contamination has . Contamination is commonly introduced from laboratory reagents, the adjacent environment and DNA extraction kits, called ‘kitome’ contamination; all can significantly drown the “true signal” from the test sample . This is a difficult problem to resolve since many contaminants are microorganisms also present in the respiratory tract, therefore, complete bioinformatic removal of contaminants may result in loss of biologically relevant taxa . The inclusion of sampling and processing controls is highly recommended to improve contaminant detection, however many paediatric studies have not reported such strategies; this may impact accuracy of findings .
Laboratories should assess their “limit of detection” – the threshold concentration at which sample DNA is overcome by contaminant DNA. This can be achieved using a “mock community” positive control- a diverse range of in vitro microorganisms found in the airways of known composition and concentration, although in lung microbiota research has rarely been employed .
Previous reports performing such approaches indicate this limit of detection may lie between 10 4 -10 6 copies of 16S rRNA, which may be the biomass of paediatric BALF samples . In addition, metagenomics may require a minimum 10 8 bacterial cells to reliably classify microbiota present within samples . Therefore, reporting of the microbial burden in samples should be a necessary component in low-biomass studies . Samples that fall below an established threshold may not be used for microbiota sequencing, improving the quality of data.
Despite the technical challenges and limitations, many studies have begun to explore the role of the lung microbiota from birth and beyond.
Lung microbiota in the first weeks of life – predictors of lung disease?
Infant host-microbiota interactions in health
The early predictors of lung health or disease in children involve ante-, peri- and post-natal factors [ Fig. 4 ]. Preterm delivery impacts normal staged lung development and early exposure to oxygen as well as other environmental insults (eg positive pressure ventilation) likely play a detrimental role. The role of infective agents is also an important consideration since preterm delivery may be triggered by in utero inflammatory or infectious events . Thus, infant host-microbe interactions likely influence longitudinal lung health .

Pattaroni et al . investigated the tracheal microbiota in ventilated preterm (n = 26) and term (n = 19) mechanically ventilated “healthy” infants in the first year of life. Gestational age had the greatest influence on lower airway microbiota .
In preterm infants, the microbiota clustered into Staphylococcus or Ureaplasma -dominated environments in caesarean-section and vaginally delivered neonates, respectively. In term infants, a more diverse microbiota colonised the trachea irrespective of mode of delivery. Beyond 30-weeks’ gestation, airway surfactant increases in phospholipid content which may create a more hospitable environment, permitting more diverse microbial interactions in the lower airways in gestationally older neonates. Interestingly, in all infants, the TA microbiota stabilised, and differences resolved, beyond the second month post-birth, with communities reflective of the lower airways in adults. Abundant genera include Veillonella , Porphyromonas , Prevotella , Streptococcus and Neisseria , with the latter two proposed as keystone genera shaping the lower airway microbiota structure in healthy infants .
Transcriptomics was also performed on TA samples from a subset of participants and appeared to indicate microbial influence on the host. Immunoglobulin A (IgA) and anti-IgA pathways were upregulated, suggesting microbial ‘priming’ of mucosal immunity in early life, or, conversely, airway mucosal immunity influences microbiota composition/colonisation . Importantly, the study did not control for BPD within the study population (40 % of preterm infants), questioning our understanding of the development “healthy”, preterm, lung microbiota in the first weeks of life.
Bronchopulmonary dysplasia
Up to half of infants born before 28 weeks gestation are at risk of developing BPD, since the canalicular, saccular and early alveolar stages of lung development can be impaired. BPD is characterised by altered alveolar development, impaired pulmonary vascularisation and chronic inflammation leading to prolonged requirement for ventilatory and/or supplemental oxygen support . Numerous risk factors have been identified but the role of lung microbiota is, as yet, unclear . Early sampling in “at risk babies” for BPD can provide insight to the initial colonisers and/or changes to lower airway microbiotas prior to established disease.
Ureaplasma and BPD
The role of Ureaplasma in the lower airways and BPD development is controversial .
Staphylococcus (68 %) and the vaginal commensal Ureaplasma (18 %), were identified as most dominant microbes in the trachea over the first 3 weeks of life. Infants who developed BPD had lower abundance of Staphylococcus and higher abundance of Ureaplasma after birth plus greater microbial community instability . In preterm infants with increased abundance of Ureaplasma , reductions in total bacterial was noted, although this may have been influenced by antibiotic therapy . Gallacher et al. collected longitudinal samples in 55 preterm infants over the first month of life (50 developed BPD), linking antibiotic use to increased abundance of members of the Mycoplasmatota (previously Tenericutes) phylum, particularly Ureaplasma and Mycoplasma , microbes not susceptible to routinely prescribed antibiotics. Furthermore, Il-6 and IL-8 levels in the lower airways remained elevated despite antibiotic treatment, suggesting a pathogenic role for these taxa with cytokine levels peaking one-week post-birth . Other studies have acknowledged the presence of Ureaplasma but failed to find associations with BPD risk . Therefore, the premature airways are likely more susceptible to Ureaplasma colonisation in vaginally-delivered infants, and commonly prescribed antibiotic therapy may reduce competition causing an increase in the relative abundance of these intracellular bacteria such as Ureaplasma and Mycoplasma in TA samples . However, a causal link between Ureaplasma abundance in early life with BPD development requires further study.
Pseudomonadota, Lactobacillus and BPD
Reduced microbiota diversity caused by increased abundance of the Pseudomonadota (previously Proteobacteria) phylum has been correlated to the development of BPD .
Stenotrophomonas (on day 1 of life) has been associated with the development of severe BPD (but not mild-moderate disease) and appears to correlate with increasing concentrations of sn -glycerol 3-phosphoethanolamine ( sn -G3PE), a cell membrane constituent, involved in glycerophospholipid metabolism and potential biomarker in BPD .
Others found levels of Enterobacteriaceae and Lactobacillus were positively and negatively linked to BPD, respectively . Individuals with lower levels of Lactobacillus in TA samples collected within 6-hours of birth went on to develop BPD, suggesting a protective role in the developing airways . In germ-free mice, introduction of Lactobacillus at the nose positively influences alveolar development and lung immunity . Further possible links between altered microbiota at birth and metabolic changes in the lower airways have been described. The airway metabolome of BPD-infants is augmented in pathways related to fatty acid-metabolism including both androgen and oestrogen generation; such findings may underlie the sex-related differences in BPD development .
However, there are important caveats to consider. There is often a lack of adequate reporting on sampling and/or processing controls . Microbial DNA positively, ( Acinetobacter , Enterobacteriaceae, Stenotrophomonas ), or inversely ( Lactobacillus ) linked to BPD can be found in laboratory reagents . Moreover, BPD is characterised by disrupted alveolarisation, therefore, tracheal microbiota may not accurately reflect the alveolar ecosystem . However, there are practical and ethical issues with repeated sampling alveolar regions in neonates .
Whilst premature birth appears to reduce lower airway microbial diversity, further studies are required to separate specific microbiota associations with health and disease in young infants. Early-life exposure to a diverse range of microorganisms has been shown to be inversely proportional to the risk of asthma development suggesting early life exposure to diverse microbes and establishment of a varied airway microbiota may have an important role in the development of lung disease .
Asthma
Asthma is very common in children, characterised by increased mucus production, reversible airway obstruction, airway inflammation and remodelling, resulting in impaired lung function. Airway inflammation in asthma is characterised by an exaggerated Th2, eosinophilic response, whilst increased Th1 and Th17 responses have been described in corticosteroid -resistant and difficult asthma . Despite asthma affecting the upper and lower airways, there is currently a lack of data relating to lower airway microbiota research in paediatric asthma. Moreover, studies have mainly examined small sample sizes .
Increased abundance of Haemophilus and Staphylococcus , and reductions in Prevotella , have been observed paediatric asthma . Others found no significant differences between children with severe asthma and disease controls, although evidence of bronchial inflammation was reported in controls . A study that incorporated healthy controls into their study design found differences in α-and-β-diversity between asthmatics and healthy controls . Asthmatic children demonstrated increased abundance of fungal Malasezzia and lactic acid-generating bacteria Streptococcus and Enterococcus coincided with reductions of an unclassified fungus and Lactobacillus . Moroever, reductions in Penicillium aethiopicum and Alternaria species were viewed in children with difficult asthma. Differences in bacterial species were observed between paeditaric and adult asthmatic sputum, although longitudinal studies are needed to stenghten these age-dependent changes of lower airway microbiota in asthma .
Another important question is how therapies impact lung microbiota in asthma (or vice-versa). Differences in the sputum microbiota of asthmatic children have been shown between those receiving steroid therapy versus dual steroid and leuktirene receptor anatagonsts, although the clinical importance of this observation is unknown .
Importantly, most paediatric asthma exacerbations are triggered by viral pathogens, in particular rhinovirus, however, there are no microbiome studies which have incorporated the role of lower airway viruses in paediatric asthma . In asthmatic adult sputum, the integration of DNA-based viruses demonstrated greater predictability of disease severity than 16S rRNA gene analyses, therefore, should be a future research aim in paediatrics .
Overall, there is a lack of data investigating relationships between lower airway microbiota and asthma. Longitudinally studies sampling the lower airways shortly after birth that follow infants over childhood would strengthen mechanistic animal work that implicates early-life microbiota interactions with asthma development . Furthermore, our understanding of how lung microbiota develops over time in children is largely derived from studies in CF.
Cystic fibrosis
CF lung disease is typified by a constant cycle of neutrophilic inflammation and infection, which, coupled with cystic fibrosis transmembrane conductance regulator (CFTR) dysfunction results in viscous secretions, impaired airway clearance and chronic infection by “traditional” CF-associated respiratory pathogens such as Pseudomonas aeruginosa . Studies utilising NGS have observed polymicrobial activity in the lungs, including Streptococcus , Veillonella and Prevotella , referred to as “non-traditional” taxa . Some suggest depleted lung microbiota diversity is tightly linked to disease progression in childhood ( Fig. 5 ) .
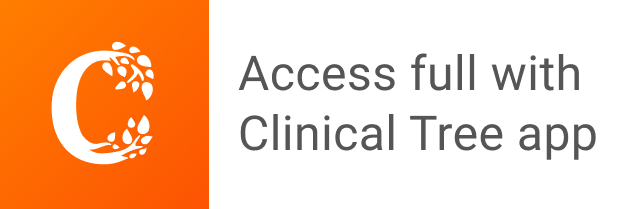