Fig. 3.1
(a) Changes of length and width of mouse cardiomyocytes during growth. Birth day set as 0. Data from embryonic day 8.5 (−11.5) to 4 days after birth taken from Hirschy et al. (2006). Data from birth to 205 days taken from Leu et al. (2001). Data from RV and LV were averaged. Plotted width is average of cell thickness and width data. (b) Electron micrograph from mouse heart showing part of a binucleate (nuc) cardiomyocyte and its connections through the intercalated disc (asterisk) to two other cells. A capillary (cap) is also indicated
The final size of cardiomyocytes in adult mammals is relatively similar from mouse to man. It is difficult to compare exactly because it depends on many factors such as age, nutrition and exercise, but a length of ~150 μm, and a width of 20 μm is common (see, for example, Bishop et al. 1979; Smith and Bishop 1985; Gerdes et al. 1992; Leu et al. 2001). Alterations in these dimensions occur in diseased hearts as will be seen later.
3.2.2 Morphology of Cardiomyocytes During Growth
There are significant changes in the morphology of cardiomyocytes during different phases of growth. At first (e.g. embryonic day 8.5 in mice) the cells are small and relatively round; a few myofibrils, which are not well aligned, surround the nucleus (Hirschy et al. 2006). Gradually, the cells elongate with a concomitant lengthening and improved alignment of the myofibrils. At birth the cardiomyocytes are long narrow elliptical cells ~50 μm in length and 10 μm in width. After birth, continued elongation and a spate of lateral growth bring the cells in a few weeks to their adult appearance, some 50 % longer and wider. They are mostly by now binucleate cylindrical cells with stepped ends (Fig. 3.1b). The ends overlap and interdigitate with several other cells (Figs. 3.1b and 3.2a). During subsequent hypertrophic growth, the structure is consolidated (Fig. 3.2c). How and where this growth, illustrated diagrammatically in Fig. 3.2b, occurs and is maintained is the major subject of this chapter.
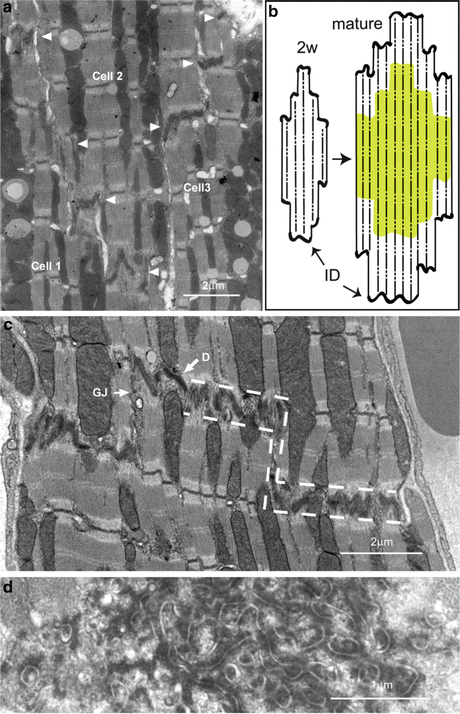
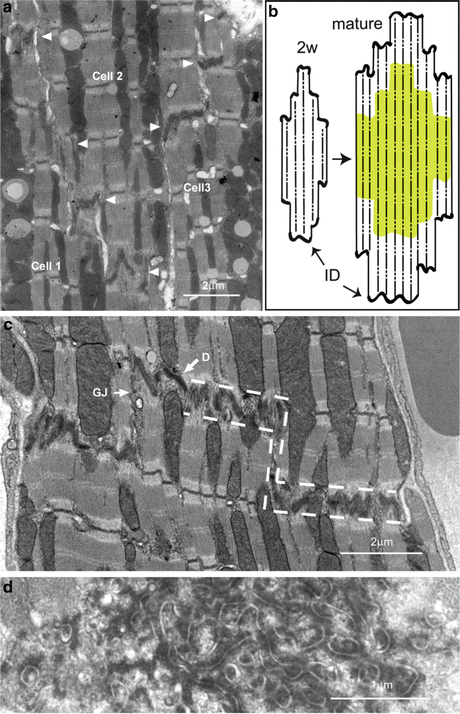
Fig. 3.2
(a) Electron micrograph of 2-week-old mouse heart showing stepped ID between three cardiomyocytes. At this age the treads of the steps (arrowheads) are narrow. (b) Diagram to show growth of cardiomyocytes from 2 week cells at the end of the hyperplastic, neonatal, stage with small lateral ID steps to longer adult cells (2+ months) with established wide ID steps. (c) Electron micrograph of a longitudinal section of 6 month mouse papillary muscle illustrating the increase in lateral width of the ID treads compared to (a). Dotted lines show outline of the area defined as the ID. (d) Transverse section through an ID tread showing the circular profile of the membrane folds. D desmosome, GJ gap junction
3.2.3 Cardiomyocyte Connections
Even at the earliest stage the myocytes are connected to each other through cadherin-rich structures, adhering junctions, found over most of their surface (Hirschy et al. 2006). The early myofibrils are attached through these junctions to the plasma membrane, and the spontaneous contraction of the cells which occurs very early in heart development sets up a pattern of forces which leads to the reorganisation and alignment of the myofibrils and the changes in morphology of the cells. The importance of these structures in heart development is demonstrated by studies such as that of the N-cadherin knockout mouse where the mouse dies in mid-gestation when the heart cells fail to adhere (Radice et al. 1997). In the early heart, the tessellated organisation of the almost round cells means that they interact with several others. As the cells elongate connections through their ends with several neighbouring cells are maintained (Figs. 3.1b and 3.2a). As the cells lengthen, the cadherin-rich junctions apparently move towards the ends of the cells (Fig. 3.2b). As the width of the cell increases these connections become wider and more substantial, and the stepped intercalated disc as it is normally described is created (Fig. 3.2b, c).
3.3 Myofibril Formation and Growth
3.3.1 Early Formation of Myofibrils
There are a number of theories about how myofibrils are formed initially (see Sanger et al. 2005). Much of the work has been carried out on cultured cell lines or isolated neonatal cells. In such myocytes or myotubes, the observations have led to the idea of premyofibrils or stress fibre-like structures which contain sarcomeric proteins but only later become full length mature sarcomeres. These in vitro studies use essentially a two-dimensional system rather than a three-dimensional one. In three-dimensional or in vivo systems, there is little evidence for these intermediate sarcomeric structures (Ehler et al. 1999, 2004). There is, however, in agreement that some kind of scaffold of Z-disc and M-band proteins is laid down first and thick and thin filaments are then incorporated. Increase in length of the myofibrils in the in vitro systems appears to be by the addition of new sarcomeres at their ends.
In early heart, in vivo, only short myofibrils with a mature sarcomere length are seen (Hirschy et al. 2006). The question arises, ‘Where are new sarcomeres added?’ The evidence suggests that, at least in the mature heart, they are added at the ends of the fibrils near the intercalated disc.
3.3.2 Evidence for Fibril Growth at the Ends of Myocytes
Evidence for the growth of muscle cells occurring at their ends is reviewed in (Russell et al. 2010). In skeletal muscle, growth occurs at the ends of the fibres near the myotendinous junction (MTJ). In early work Williams and Goldspink (1971) labelled young rapidly growing skeletal muscle with tritiated adrenaline to follow where actin is deposited and found it accumulated at the ends of the fibres. This was supported by the observation in the electron microscope of copious ribosomes in these areas and bundles of thick filaments (no Z-discs) and narrow myofibrils at the periphery of the muscle. Dix and Eisenberg (1990) using mRNA detection of slow myosin heavy chain on stretched stimulated TA muscle in the rabbit which normally expresses the fast isoform, found a large accumulation of the slow isoform at the MTJ. In addition, in contrast to the control muscle where the regular sarcomeric structure is present up to the edge of the MTJ, in stretched muscle, bundles of filaments and thin myofibrils in a sea of new T-tubules, ribosomes and mitochondria were seen at the MTJ.
There is a constant turnover of sarcomeric proteins in muscle fibres (Willis et al. 2009). However, evidence for formation of new sarcomeres within the body of the fibre is seen only after eccentric exercise. Here, local changes in the distribution of desmin and Z-disc proteins such as α-actinin are seen and have been attributed to myofibril remodelling after damage inflicted by sudden large transient stretches (Yu et al. 2004). Such repair sites are not observed in control muscle. Similar repair or addition of sarcomeres has been seen in cardiomyocytes as a result of a major 10 % stretch (Yu and Russell 2005). In this case the stretch was permanent so the cells had to generate a number of additional sarcomeres in order to normalise the sarcomere length. The rate at which this occurred was about one sarcomere (2 μm) per hour much greater than normal even in early heart growth (see Sect. 2.1). In addition to fibril remodelling, the stretch also generated substantial changes in the morphology of the ID suggesting the involvement of this region in growth. This observation is supported by recent evidence that there are significant changes at the ID correlated with growth brought about by volume overload in the rabbit heart (Yoshida et al. 2010). In addition, evidence for sarcomere addition at the ID has been seen in both normal heart and those suffering from dilated cardiomyopathy (DCM) (Wilson et al. 2014). In order to understand this, and indeed the more extensive role of the ID, we have to look more closely at its structure and its relationship with the myofibril.
3.4 Structure of the Intercalated Disc
3.4.1 General Organisation
What exactly do we mean by the intercalated disc? The main body of the cardiomyocyte comprises the myofibrils interspersed with columns of mitochondria extending from one end of the cell to the other (Fig. 3.1b). These are held, together with the sarcoplasmic reticulum and t-tubules, within a cytoskeletal network of proteins such as desmin, the whole being anchored to the extracellular matrix (ECM) at the lateral plasma membrane by the costameres (Granger and Lazarides 1978; Craig and Pardo 1983; Pardo et al. 1983) (Fig. 3.2c). The ID is seen in the light microscope as a high density junction between the end of one heart muscle cell and the next. With the advent of electron microscopy, it became clear that it was a complicated structure with regions of highly folded membrane of variable amplitude (compare Fig. 3.2a, c). Here, we adopt the definition of the ID given by Forbes and Sperelakis (1985). It includes the membrane folds and their axial connections as well as the material within the folds delineated by the dotted lines in Fig. 3.2c.
The structure of the ID has been beautifully described in several excellent electron microscope investigations (see, for example, Fawcett and McNutt 1969; Forbes and Sperelakis 1985). The general form of the ID is a stepped structure. The treads of the steps are lateral stretches containing folded membrane (Fig. 3.2). In transverse section the folds (or peaks) are seen as circular sections so that the ID tread membrane can be thought of as having a structure like an egg box (Fig. 3.2d). Within the folds, the thin filaments from the terminal sarcomeres of the myofibrils run to the membrane (Fig. 3.3a). Since the myofibrils take up approximately two-thirds of the cross-sectional area of the cell, much of this membrane is associated with actin adhering junctions. However, since the mitochondria occupy typically most of the other third of the cell a significant portion of the ID structure is available for other functional regions such as desmosomes, signalling domains and possible some that relate to mitochondria. The risers of the ID steps are axial and usually have a length of one or more sarcomere lengths. Often in the mouse these steps contain gap junctions (Fig. 3.2c). It is worth noting that the close association of the membrane of the neighbouring cells at the ID means that there is little or no ECM here.
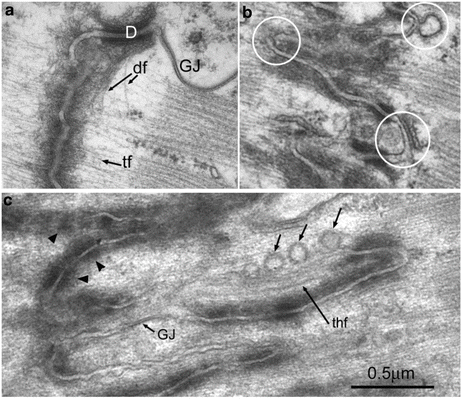
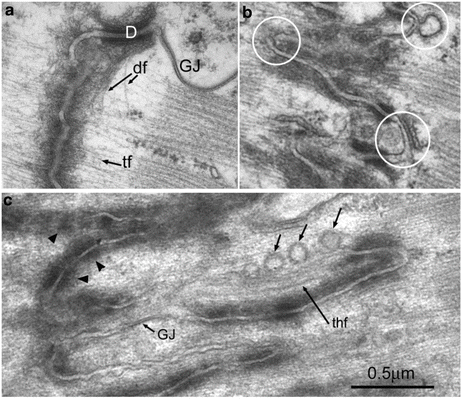
Fig. 3.3
Electron micrographs of longitudinal sections through mouse heart showing ID folds of different amplitudes. (a) Thin filaments (tf) run into dense protein plaque at the ID membrane. Desmin filaments (df) can be seen leaving a desmosome (D). GJ gap junction. (b) ID of intermediate amplitude. Circles show the tops of the folds which are devoid of CJ plaque which is associated with the sloping sides of the folds. One of the circles rings a fold with a junctional SR vesicle closely opposed to the ID membrane. Another shows a coated vesicle apparently combining with it. (c) ID of extreme length shows long regions of uncoated membrane some of which is associated with vesicles. Arrowheads show regions where CJ plaque is fragmenting. GJ small gap junction, thf thick filament within a long fold
3.4.2 ID Membrane: Domains and Connections
Desmosomes
Desmosomes are very dense junctions, often found between cardiomyocytes where the mitochondria columns end (Figs. 3.2c and 3.3a). The role of the desmosome is to weld the plasma membranes of two cells together. In addition, they attach through desmoplakin to the main intermediate filament in muscle, desmin. In Fig. 3.3a, the desmin filaments are seen leaving a desmosome (see also Forbes and Sperelakis 1985). They run across the fibril and appear to weave their way across the tops of the ID peaks. As well as being found at high concentration at the ID, these intermediate filaments are a cytoskeletal component at the costameres, where the lateral PM is attached to the Z-disc, and wind their way at the Z-disc level around the fibrils across the cell (Granger and Lazarides 1978). This distribution can be seen by immunofluorescence in Fig. 3.4. It is clear that at the ID desmin collocates closely, although not exactly, with the adhering junction protein cadherin (Fig. 3.4a). The principle proteins found in cardiomyocyte desmosomes are shown in Fig. 3.5. The arrangement of the proteins within the structure has been characterised by North et al. (1999). The desmosomal cadherins, desmoglein2 and desmocollin2, are transmembrane glycoproteins which link cells through their N-terminal extracellular domains. This binding is partly calcium dependent and a high level of extracellular calcium is required for strong binding and physiological function (Dusek et al. 2007; Garrod and Chidgey 2008). The intracellular C-terminals of the cadherins bind through the armadillo proteins plakoglobin and plakophilin to desmoplakin which in turn binds to desmin filaments.
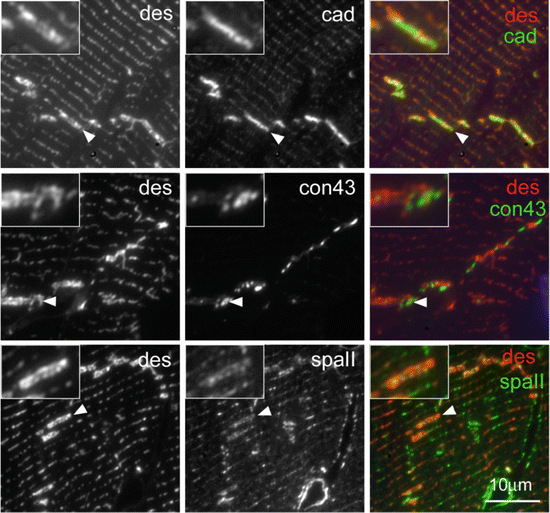
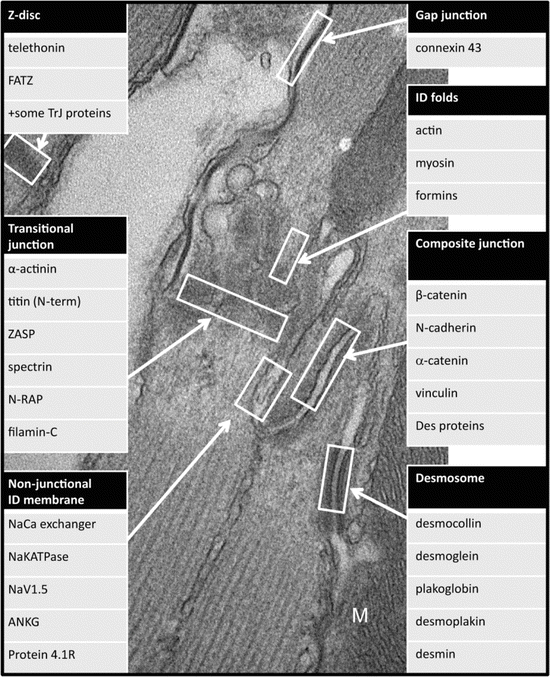
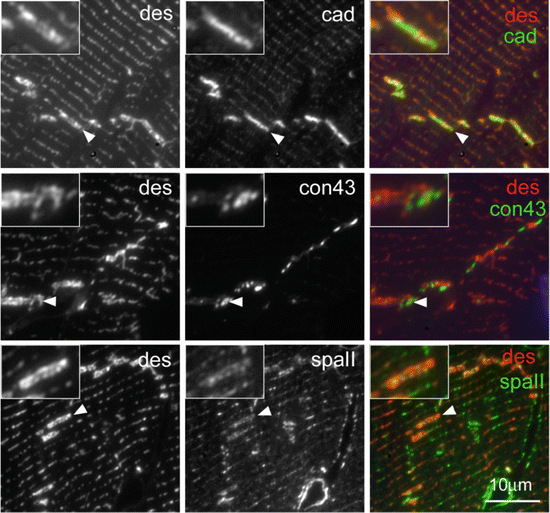
Fig. 3.4
Immunofluorescence of desmin and other ID proteins, N-cadherin, connexin43 and aIIspectrin in thin cryosections of mouse papillary muscle. (a) desmin (des, red) is strongly present in the ID (arrow) and at the Z-disc level in the rest of the cell. Pan cadherin (cad, green) localises closely but not exactly with it at the ID. (b) Connexin43 (con43, green) does not colocalise with desmin since it is found mostly in the axial risers of the ID and not the lateral treads. (c) αII spectrin (spaII, green) is seen at the ID treads and the Z-disc level but does not colocalise with desmin in either place. Inserts show at 2× magnification the ID indicated in the figure
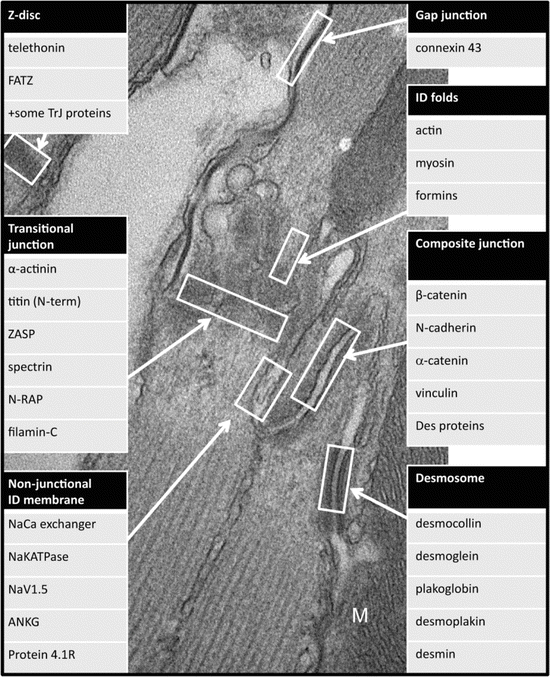
Fig. 3.5
Electron microscope image showing the different regions within the ID and the proteins associated with them
Gap Junctions
At gap junctions the membranes are closely apposed, bound together by pore proteins (Fig. 3.3a). Hexamers of the protein, connexin, align with those in the other cell creating connexons. These form a tightly packed array which allows ions and small molecules to pass across the membrane transmitting and propagating the electrical signal that keeps the heart beating regularly. In adult heart the main isoform is connexin43 (Fig. 3.5). In mice these junctions are generally found on the axial steps of the ID membrane as seen in Fig. 3.2c although there are small patches embedded in the ID folds (Fig. 3.3c). There is little overlap between gap junctions on the risers and the other junctions (Pinder et al. 2012; Wilson et al. 2014). This separation can be seen in Fig. 3.4b where the location of connexin43 is compared to desmin by immunofluorescence. Further, it has been shown that gap junctions relocate to the ends of the cells during development with a slower time course than the adhering junctions and desmosomes (Angst et al. 1997). There is thought to be a buffer zone around the gap junction patches rich in the tight junction protein, zonula occludens-1 (ZO1) (Hunter et al. 2005). However, it is otherwise unclear what locates the gap junctions in their place. For a review of connexin interactions, see (Herve et al. 2007).
Composite (Adhering) Junctions
At high magnification in the electron microscope, the path of the thin filaments from the terminal sarcomere into the ID folds is clear (Fig. 3.3a). They feed into the dark protein-rich plaque at the adhering junction (Forbes and Sperelakis 1985). The orientation of the thin filaments is such that it is their barbed ends that are at the membrane (Yamaguchi et al. 1988). The junctional protein is found on the sloping sides of the folds so that the actin filaments come in at an oblique angle to the membrane (Fig. 3.6). This geometry applies shear stress as opposed to strain at the membrane which has been shown to give more strength to the junction (Tidball 1983).
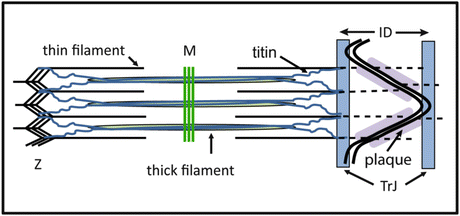
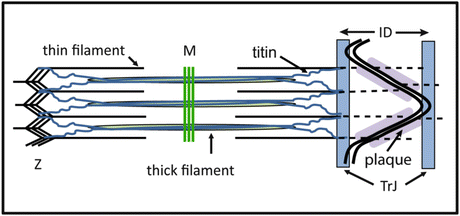
Fig. 3.6
Diagram showing the arrangement of the thin filaments in the intercalated disc and the transitional junction. Thin filaments run from the last half sarcomere into the CJ plaque at the ID membrane. At a position equivalent to the Z-disc, a region rich in Z-disc proteins including N-terminal titin is found. This position is equivalent axially to that of the tops of folds of the ID membrane. The membrane tops and the ‘Z-disc’ region is defined as the transitional junction (TrJ)
The main protein components of the ID plaque are those of adherens junctions (AJ) and desmosomes (Fig. 3.5) (Borrmann et al. 2006; Franke et al. 2006). AJs are similar in organisation to desmosomes. The transmembrane protein, N-cadherin, is bound to the armadillo proteins, p120, β-catenin and α-catenin. This complex is connected through vinculin, an actin binding protein, to the thin filaments. It seems that in the very early embryonic heart, desmosomes and adherens junctions are separate but by birth they are intimately combined (Pieperhoff and Franke 2007). The resulting junctions have been called areae compositae (Borrmann et al. 2006) or hybrid AJs (Li and Radice 2010). Here they will be called composite junctions (CJ). It is not clear what the functions of the desmosomal proteins are in the CJ. There are apparently obvious desmosomes where desmin filaments attach as described above, but it is not obvious that these filaments penetrate the dense plaque of the CJ and bind. The distribution of desmin with respect to N-cadherin at the ID seen in Fig. 3.4a suggests that there is not a strong correlation between the two. Possibly, the close association of AJ and desmosome gives rise to a stronger junction that is necessary to support the high tensions found in contracting heart. Of the proteins involved, one, plakoglobin (γ-catenin), is known to operate in both types of junction and can replace β-catenin in the adherens junctions (Zhurinsky et al. 2000). Another desmosomal protein, plakophilin-2, has been shown to interact with a specific region of αT-catenin, its adhesion modulation domain (Goossens et al. 2007). These two interactions might be the means of knitting the adherens and desmosomal proteins into a specialised domain.
Non-junctional Membrane
Apart from the structural junctions described above, there are a number of ‘bare’ or unspecialised membrane regions in the ID (see Forbes and Sperelakis 1985). These probably comprise different types of domains that may play a number of different roles. Among these areas are the tops of the folds (Fig. 3.3b) and part of the axial risers devoid of gap junctions (Fig. 3.5). In addition, extra membrane is generated when big amplitude ID folds are formed (Fig. 3.3c). Estigoy et al. (2009) have identified some 200 proteins at the ID using immunohistochemical data on the Human Protein Atlas (HPA) website, ExPASy (website) protein binding data and published papers on IDs. A large number of these are not obviously associated with the junctional domains already described.
Two primary cytoskeletal complexes are known to underlay and support membranes. One based on dystrophin and the other on spectrin. Both are found at the lateral plasma membrane in the cardiomyocyte, but only spectrin is seen at the ID (Stevenson et al. 2005). Several components of the spectrin complex have been located there, including αII spectrin, βII spectrin, ankyrin-G and proteins 4.1R and 4.1G (Mohler et al. 2004; Bennett et al. 2006; Pinder et al. 2012). The distribution of two of these at least, αII spectrin and 4.1R, does not overlap with β-catenin or connexin43, in support of their presence on the non-junctional membrane (Bennett et al. 2006; Bennett 2012; Pinder et al. 2012; Wilson et al. 2014). 4.1R is a multivalent protein stabilising the actin/spectrin interaction and interacting through its FERM domain with membrane signalling proteins (Baines et al. 2014). Ankyrin-G also links spectrin with membrane bound signalling proteins and has a pivotal role in locating the sodium voltage channel NaV1.5 at the ID (Mohler et al. 2004).
3.4.3 The ID as Z-Disc: The Transitional Junction
Tension Transducer
Myofibrils reach to the edge of the ID but there is no Z-disc density at the ID side of last sarcomere. Since myofibrils in neighbouring cells connect through the ID, it has often been said that the ID acts as a ‘Z-disc’. However, the Z-disc has many functions and two of these, at least, are spatially separated at the ID. A principle role is to connect the thin filaments from one sarcomere to the next and to transmit the tension along the myofibril. The CJ with its dense protein complement fulfils this role. The protein complex present, including vinculin and α-catenin, are involved in tightly binding the thin filament to the membrane and use cadherins to transduce the tension across the cell membranes to the next myofibril (Fig. 3.6).
Titin Anchor and Passive Tension
A second role of the Z-disc is to anchor titin molecules. The giant titin molecule runs from the Z-disc, through the I-band, along the thick filament in the A-band ending in the M-band (Fig. 3.6) (Kontrogianni-Konstantopoulos et al. 2009). I-band titin carries the passive tension in the sarcomere and thus maintains the ordered arrangement of filaments (Horowits 1992). This is effective because the I-band thin filaments are all the same length. However, the thin filaments from the terminal half sarcomere near the ID are all different lengths since they insert in the membrane at different points on the slope of the fold. If titin from the terminal half sarcomere was inserted at the membrane end of the thin filaments, each molecule would exert a different passive tension on the thick filaments and there would be disorder in the A-band. However, it is clear that the ordered arrangement of the sarcomeres is maintained right to the edge of the ID. Furthermore, the sarcomeric thin filament proteins tropomyosin and troponin finish at this point and are not found in the ID folds. This led to the definition of a transitional junction (TrJ), a region at the margin between myofibril and ID that behaves like a Z-disc with respect to titin although it has no Z-disc density (Fig. 3.6) (Bennett et al. 2006).
The Transitional Junction
The proteins associated with the TrJ have a characteristic distribution. They show themselves as a doublet about the ID with an axial separation corresponding to the amplitude of the ID folds. Several sarcomeric Z-disc proteins are to be found at this position. These include N-terminal titin (Fig. 3.6), α-actinin and ZASP [(cypher/oracle; Faulkner et al. 1999; Zhou et al. 1999; Passier et al. 2000) Bennett et al. 2006; Bennett 2012]. How they maintain their location is unknown. Compared to the Z-disc there is a lack of mass at the TrJ. This is partly due to the absence of overlapping thin filaments of the opposite polarity. In addition, some Z-disc proteins are absent (Bennett 2012; Wilson et al. 2014). The two known to be missing are telethonin (T-cap) which binds to the N-terminal Ig domains of two titin molecules (Valle et al. 1997; Gregorio et al. 1998) and FATZ (calsarcin/myozenin) which is a ZASP/titin binding protein (Faulkner et al. 2000; Takada et al. 2001; Frey and Olson 2002). Their location at the Z-disc may rely on the antiparallel arrangement of thin filaments.
Other, non-Z disc, proteins exhibit the same doublet distribution across the ID. N-RAP is a nebulin-like repeat protein with five nebulin super repeats suggesting it binds to actin/tropomyosin filaments (Luo et al. 1997; Zhang et al. 2001). It may replace the Z-disc protein, nebulette, at the TrJ. Another protein with the doublet spacing is αII spectrin which has been localised at the tops (peaks) of the ID folds (Fig. 3.4c) (Bennett et al. 2006; Wilson et al. 2014). This suggests that there is a membranous region associated with the TrJ and that there is a close structural and functional connection between them. A protein that may link between the spectrin-rich membrane folds and the myofibrillar TrJ is filamin C which exhibits the doublet distribution (van der Ven et al. 2000). Filamin C is known to bind between membrane proteins, sarcoglycans, present at the ID and the ID proteins, N-RAP and Xin (van der Ven et al. 2006).
The ID Thin Filaments
Within the ID folds themselves, there is evidence that the actin isoform in the thin filaments is not cardiac α-actin (Bennett et al. 2006) but possibly β-actin (Benz et al. 2013). Other proteins have been associated with these filaments in the ID (Fig. 3.6). One is formin, the protein that aids barbed end linear actin polymerisation (Randall and Ehler 2013); the muscle specific FHOD3 is strongly represented at the ID (Iskratsch et al. 2010), and the ubiquitously expressed FHOD1 has been shown to interact with α-catenin (Kobielak et al. 2004). The ID formins may therefore be located at the CJ and involved in the elongation of thin filaments there. MENA/VASP also has an important role in actin polymerisation. It interacts with αII spectrin and has been found in the ID (Eigenthaler et al. 2003; Benz et al. 2013). Non-muscle myosin II is also located in the ID (Takeda et al. 2000).
3.5 The ID/Transitional Junction in Longitudinal Growth
3.5.1 Variation of ID Amplitude
We have seen that the increase in length of cardiomyocytes is likely to occur at the end of the cells, at the ID. An interesting feature of the ID is that the amplitude of the membrane folds varies (Figs. 3.2 and 3.3). Measurements of its amplitude show that it can vary from 20 nm to 2 μm with an average of 0.5 μm (Wilson et al. 2014). A myocytes 100 μm in length could therefore grow by about 1–2 % simply by increasing the ID amplitude. The heart grows as a result of local pressures to pump more blood. This is likely to be a small incremental effect over time so the ability of the cells to slowly change their length will be important. Equally so will be the ability to reverse the process when demands on the heart are reduced (Frenzel et al. 1988).
Increase in the ID amplitude involves both the elongation of the thin filaments and an increase in the surface area of the ID membrane. As described above, proteins that aid thin filament length extension, formins and MENA/VASP, are present in the ID. As the thin filaments extend the membrane also extends. In a normal muscle the ID amplitude is on average ~0.5 μm. A crude estimate suggests that the area of the ID membrane at this amplitude is about 1.6× that at its smallest amplitude. An increase in amplitude to 2 μm involves a ~5-fold increase in membrane area. Perhaps not surprisingly, high amplitude IDs are often the site of multiple vesicles (Fig. 3.3c). Both coated vesicles and calveolae have been previously described at the ID (Fawcett and McNutt 1969; McNutt and Fawcett 1969).
At higher amplitudes, there is a difference in the appearance of the CJ regions. At low amplitude the CJ covers essentially all the membrane slopes (Fig. 3.3a). As the amplitude increases, the CJ seems to fragment and smaller lengths of plaque are seen (Fig. 3.3b, c). This is perhaps not surprising since there are a fixed number of thin filaments coming from a sarcomere. We might assume that a single thin filament will require a given amount of plaque complex for a firm attachment to the membrane. Consequently, a smaller proportion of the membrane of high amplitude IDs will be needed for this function. As a result of the plaque breakup, there is now a significant amount of ‘bare’ membrane which could lead to an increase in signalling domains.
3.5.2 Sarcomere Insertion
It is clear that there is a natural limit to the amplitude of the ID folds. They rarely grow longer than 2 μm. This corresponds to one sarcomere length and suggests that at this point, a new sarcomere could be inserted. Evidence for this comes from the work of Yoshida et al. (2010). Working with a model of volume overload by arterio/venous fistula in rabbits, they showed that there was hypertrophic growth and that the myocytes grew every 2 days by two sarcomeres. In the course of this time there was change in the ID morphology. In particular, starting from a normal ID amplitude the ID grew more convoluted until it reached a size of ~2 μm. It then returned to its original size. During this time it went through several morphological stages one of which the authors attributed to the insertion of a sarcomere on each side of ID. On removal of the overload the process reversed.
Wilson et al. (2014) have looked for evidence for this happening during normal growth. Since the normal rate of growth in the mouse heart is something like one sarcomere a week and it has been shown that sarcomeres can be inserted in neonatal rat cardiomyocytes at the rate of 1 per hour, it might be a rare event to capture in normal growth. In high amplitude folds in IDs, sarcomeres are seen occasionally (Fig. 3.7b). Furthermore, it was sometimes possible to see isolated A-bands, bundles of thick filaments, or single filaments within these folds (Figs. 3.3c and 3.7a). Interestingly there was no Z-disc associated with these partial structures. They were reminiscent of the nascent filament assemblies described at the ends of skeletal muscle fibres near the MTJ in young rapidly growing animals or after stretch (Williams and Goldspink 1971; Dix and Eisenberg 1990).
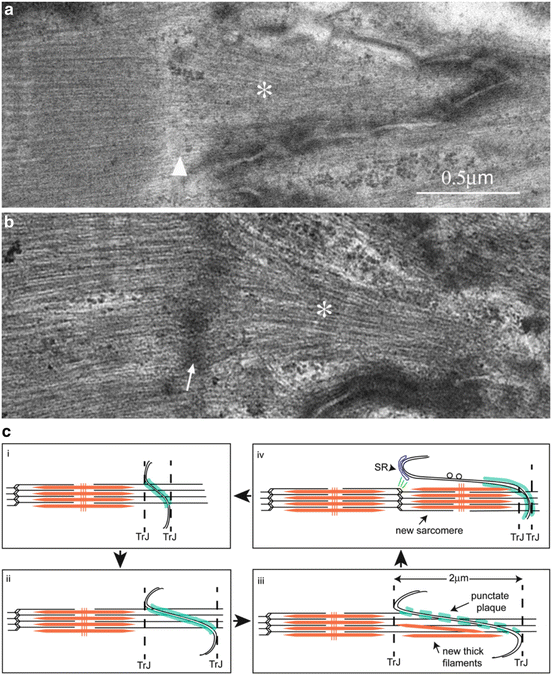
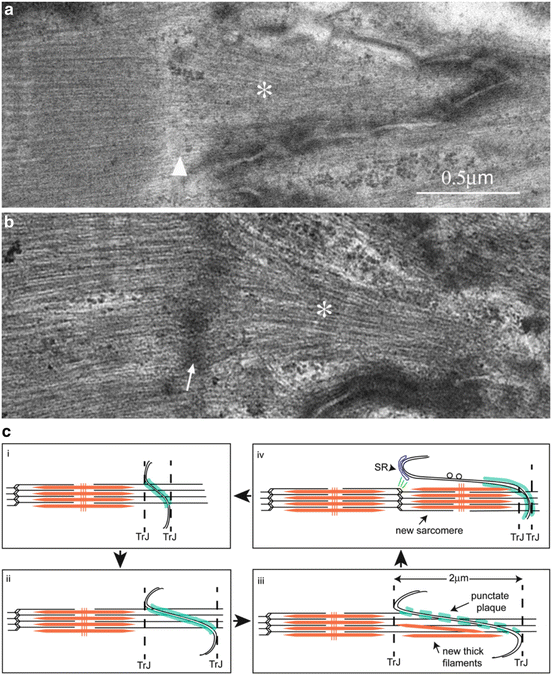
Fig. 3.7
Evidence for sarcomere insertion at the ID. (a) In mouse heart an A-band (asterisk) is seen within the fold of an ID but there is no Z-disc (arrowhead) separating it from its neighbouring sarcomere. (b) The insertion of a sarcomere (asterisk) in a fold in the ID complete with a Z-disc (arrowed) in human heart. (c) Diagram to show stages of new sarcomere formation in the heart. (i) ID with small amplitude. (ii) ID folds increase in amplitude to allow cell growth and CJ plaque (shown in turquoise) becomes punctate (iii). (iii) At 2 μm, thick filaments and (iv) whole sarcomeres are incorporated within a membrane fold. Vesicles are found on long stretches of uncoated membrane. The new Z-disc is associated with the SR at the top of the fold that may bud off to become a t-tubule. A new TrJ is established at the end of the new sarcomere. Parts of figure taken from Wilson et al. (2014) with permission
Since the long folds are symmetrical with respect to the neighbouring cells the insertion of sarcomeres on both sides of the ID are possible. But it is not clear that both fibrils that meet at the ID will grow.
3.5.3 Mechanism of Sarcomere Addition
These observations suggest a possible mechanism of ID growth and sarcomere addition in the ID (Fig. 3.7c) (Wilson et al. 2014). The TrJ is already a proto Z-disc containing many of the proteins necessary for Z-disc formation. In addition, there is continual turnover of sarcomeric proteins throughout the myocyte so the materials would be available (Willis et al. 2009). When the ID amplitude reaches a length of ~2 μm, thick filaments begin to appear in the ID fold. The proteins could be made in the ID region since ribosomes are found here or brought in the vesicles observed in the long ID folds. Alternatively, thick filaments could be transported in from elsewhere along microtubules as previously reported (Pizon et al. 2002). It certainly seems that the thick filaments are organised into bundles at the ID. This would involve the cooperation of M-line proteins, titin, myomesin and obscurin (Van der Ven et al. 1999). The new Z-disc based on the TrJ would require severing the old thin filaments at the TrJ and production of a new set of thin filaments of opposite polarity. Horowits and his colleagues have shown that N-RAP is involved in Z-disc formation (Manisastry et al. 2009). They suggest that dimers of N-RAP could lead to antiparallel organisation of thin filaments at nascent Z-discs (Crawford and Horowits 2011). The presence of N-RAP at the TrJ could therefore facilitate the formation of a new Z-disc there. As the Z-disc matures N-RAP apparently dissociates presumably to make way for nebulette together with the other Z-disc proteins, telethonin and FATZ. Telethonin, in particular, is known to be one of the last proteins to appear at the maturing Z-disc.
3.5.4 SR Extension and T-Tubule Addition
Inserted sarcomeres are surrounded by long tongues of membrane which would need to break up and be reabsorbed (Yoshida et al. 2010). It has been seen that peripheral junctional SR is often present at the top of the ID folds (Fig. 3.3b). Also, there are membrane associated proteins such as spectrin and myofibril/membrane bridging proteins such as filamin which are also found within the myocyte at T-tubule/Z-disc locations (Kostin et al. 1998). Possibly the tops of the ID folds together with the associated SR are involved in the formation of new t-tubules and SR at the new Z-disc (Fig. 3.7c).
< div class='tao-gold-member'>
Only gold members can continue reading. Log In or Register a > to continue
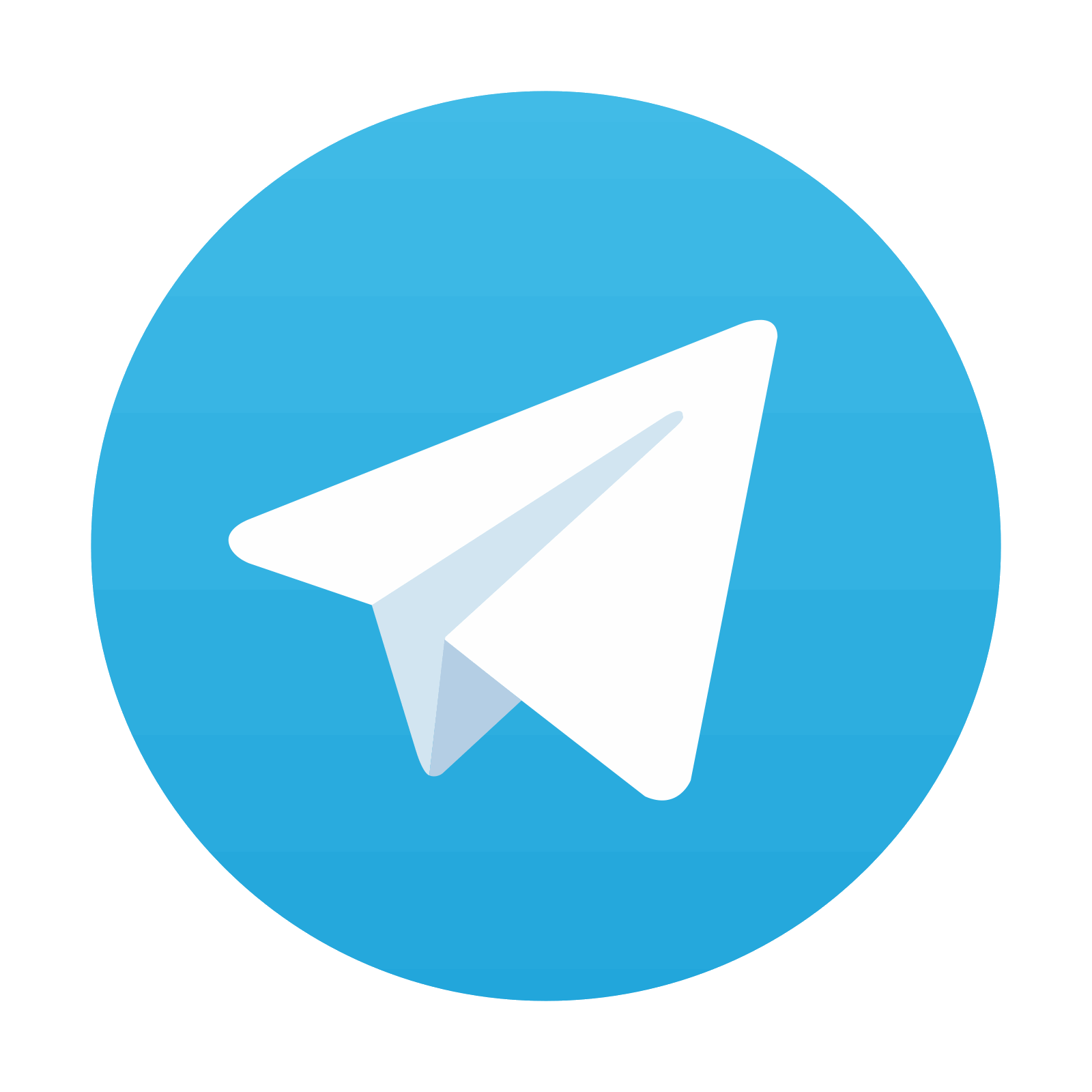
Stay updated, free articles. Join our Telegram channel
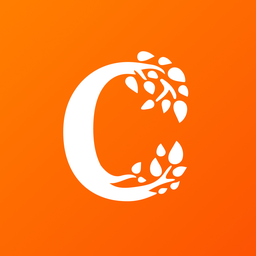
Full access? Get Clinical Tree
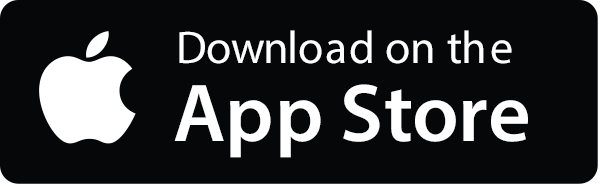
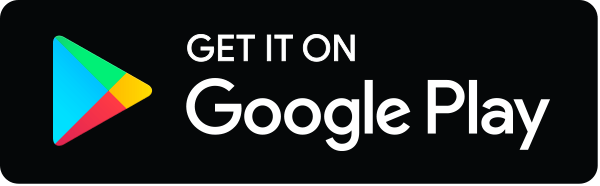