The heart is the engine that powers life, and the coronary circulation represents the fuel pipes, providing blood with oxygen and other nutrients to keep the heart beating. Under normal physiologic circumstances, there will always be an equilibrium between oxygen demand of the myocardium and oxygen supply provided by the blood flow in the coronary arteries. There is an ingenious regulation system in the coronary circulation to maintain this equilibrium, called autoregulation. Due to the enormous reserve of the coronary circulation to provide blood to the myocardium, early stages of coronary atherosclerosis and narrowing in the coronary arteries will hardly be noticed, and if the coronary arteries become more severely narrowed, complaints will only occur in situations where the oxygen demand is increased, such as physical exercise or stress. Under those circumstances, myocardial ischemia will present itself by a characteristic pain or unpleasant sensation in the chest, arms, neck, or back, called angina pectoris. However, under resting circumstances, blood flow in the coronary circulation can be kept sufficient for a long time despite the presence of important narrowing.1
For those reasons, it is important to realize that in assessing the coronary circulation, it is not the coronary blood flow at rest that should be studied as a measure for the severity of coronary artery disease, but the maximal achievable blood flow as can be provoked by maximum exercise or vasodilatory stimuli like adenosine. Stated in another way, the degree to which maximum blood flow in the coronary circulation is decreased determines the exercise level at which angina pectoris occurs.
Also, in case of acute coronary syndromes, fundamentally the same principles hold true. However, in those situations, a rapid and sharp decrease of coronary blood flow usually occurs due to plaque rupture and/or thrombosis, often superimposed upon preexisting lesions.
It should be realized that the severity of disease on the coronary angiogram has only a poor correlation with the degree to which coronary blood flow is decreased. In other words, it is difficult to assess the physiologic significance of a coronary artery stenosis from the coronary angiogram.2,3 Nevertheless, the angiogram is important because in performing coronary interventions, it is a road map for the interventionalist to manipulate with wires and other equipment and to place the stent(s). In this chapter, the structure of the coronary circulation is discussed, followed by the regulation of coronary blood flow. There is a brief discussion on the development of atherosclerosis and the physiologic methods to detect this in early and later stages. Some words are also spent on coronary stenoses and myocardial ischemia and the consequences of ischemia for the heart. This is followed by the paramount question why functional testing is important, leading to the concept of fractional flow reserve. Some physiologic aspects on background and features of fractional flow reserve are briefly discussed. The clinical use of fractional flow reserve is discussed later in Chapter 24. Finally, some specific manifestations of coronary artery disease and physiologic methods for how to address these are discussed.
In most mammalians, the coronary circulation consists of 2 blood vessels originating from the proximal aorta. In humans, these coronary arteries have a diameter of approximately 2.5 to 4.0 mm and run as a kind of corona around and across the heart. Just like all other arteries, these coronary arteries have a layered structure with an intima, media, and adventitia. It is important to realize that under normal circumstances, the resistance of the coronary artery to blood flow is negligible, even at maximum hyperemia. From the epicardial coronary arteries, perforating branches enter the myocardium, and within the myocardium, these divide further into smaller vessels, called arterioles, with a diameter between 100 and 400 μm. Around these arterioles, smooth muscle cells are located in groups and are able to vary the resistance of the arterioles over a large domain (Fig. 6-1).
FIGURE 6-1
Schematic representation of the coronary circulation. The coronary artery splits up into arterioles with a diameter of 100 to 400 μm, which further divide into the capillary bed. At the entrance of the arterioles, muscular sphincters are located that can vary the resistance of the arterioles by at least 500%. Under resting conditions, the sphincters will be constricted, and if a higher blood flow is needed in response to higher oxygen demand (as in exercise), the sphincters dilate. In that way, at an equal perfusion pressure, myocardial blood flow can increase by at least 500% in healthy young individuals. In case of a moderate stenosis, an additional epicardial resistance occurs, and as a compensation, the arteriolar sphincter partially dilates even under resting conditions in order to maintain a total resistance in the bed equal to R. Consequently, during resting conditions, no noticeable effect of the epicardial stenosis is observable. However, during situations of increased demand (eg, exercise) the compensatory vasodilator reserve of the sphincters is already decreased and the maximal achievable blood flow in the coronary artery and myocardial bed is decreased accordingly. Therefore, myocardial ischemia and angina pectoris may occur during exercise. In case of a very severe stenosis in the epicardial coronary artery (lower pictograms), the epicardial stenosis can be so tight that the arteriolar sphincter needs to dilate completely to compensate the additional resistance in the coronary artery. This means that no reserve at all is left anymore. In such a case, even minimal increase in oxygen demand will result in angina pectoris. With further increase of stenosis severity, frank ischemia at rest will occur, resulting in unstable angina or an acute coronary syndrome. (From Pijls NHJ. Coronaire fysiologie en myocardischemie. In Van der Wall EE, Van de Werf F, Zijlstra F. Cardiologie. Houten, the Netherlands: Bohn Stafleu van Loghum, 2008, with permission of Springer.22)

For the purpose of simplicity, these smooth muscle cells around the arterioles are indicated as a kind of sphincter. In reality, the muscle cells are more equally distributed along the arterioles. The resistance of these arterioles can vary in healthy individuals by at least 500%, which means that under normal circumstances, maximum blood flow can be at least 5 times baseline blood flow. This ratio between maximum blood flow and baseline blood flow is called coronary flow reserve (CFR), as will be discussed more extensively later. The arterioles split up into the capillaries, in analogy to any other organ in the human body. The capillary network of the heart is very dense, and every myocyte is next to a capillary. Finally, the capillaries collect into small venules and collect further into veins, which either drain directly into the heart chambers (Thebesian veins) or collect in 1 large vein, the coronary sinus, with a diameter of 5 to 10 mm and ending in the right atrium.
Coronary blood flow equals approximately 3% to 5% of total cardiac output and varies from 200 mL at rest to approximately 1 L per minute at maximum exercise in healthy young people. It is important to realize that in contrast to most other organs in the human body, oxygen extraction from the blood in the coronary circulation is very high and that oxygen saturation in the coronary sinus is relatively low (30%-40%). This means that in the coronary circulation increase in oxygen consumption by the myocardium can only be achieved by an increase of blood flow and not by further extraction of oxygen. This issue will be discussed later in this chapter and in the other chapters.
Under baseline circumstances, the oxygen consumption of the myocardium is 0.7 to 1.3 mL O2/min/g of tissue, and under maximum hyperemic circumstances, this increases to 3 to 6 mL O2/min/g.
During exercise, heart rate and contractility will largely increase and lead to an increase in oxygen consumption; in addition, afterload will increase, thereby further increasing the oxygen demand and consumption of the heart. To match this tremendously increased demand, a wonderful regulation mechanism has been designed by nature, called autoregulation, which is further explained in Figure 6-1.
Collaterals are small blood vessels that can connect perfusion territories of different coronary arteries. The diameter of collaterals can vary from 10 μm to >1 mm. In the latter case, the collaterals are visible on the coronary angiogram. Collaterals can either be preexistent or develop as a response to myocardial ischemia. Repetitive ischemia is a necessary condition and the most potent stimulus to develop collaterals.
Collaterals can be either subendocardial or subepicardial. In the first case, the process underlying collateral development is often angiogenesis, and such angiogenetic collaterals only exist in a 1-layered rudimentary vessel structure. Subendocardial collaterals are often nicely observed running through the septum and connecting the septal branches of the left anterior descending artery and the posterior descending branch of the right coronary artery. In the case of subepicardial collaterals, these are often preformed (with a large variation between different individuals), have a 3-layer structure like a normal small artery, and are often very tortuous (corkscrew). This type of collaterals can often be seen across the apex connecting the distal right coronary artery and the left anterior descending artery, or running from the right ventricular branch of the right coronary artery to the left coronary artery, or even running across the atrium.
Collaterals are important because in a number of patients collaterals provide sufficient blood flow to maintain oxygen supply at rest even in case of a severe stenosis or total occlusion of the recipient coronary artery belonging to the collateral-dependent territory. The extent of collaterals and the intrinsic aptitude to develop them largely vary from one person to another and are also species dependent. In humans, in case of repetitive ischemia of the myocardium, collaterals may develop and reach a kind of maximum in most patients after approximately 3 months.
Autoregulation is the mechanism by which oxygen supply and demand of the myocardium are matched to each other both under physiologic and pathologic circumstances (see Fig. 6-1). It is important to realize that the arteriolar sphincters can vary the resistance over a large range.4 If these sphincters are completely dilated, resistance in the coronary circulation decreases by roughly 500%, which means that at an equal perfusion pressure, the reserve of coronary blood flow is also 500%. Under normal circumstances, the perfusion pressure in the coronary circulation is the difference between the aortic pressure and the central venous pressure (in normal circumstances, 0-5 mm Hg). If no coronary stenosis is present, the pressure in the distal coronary artery at the entrance of the arterioles equals the aortic pressure because there is no decline of pressure in a normal coronary artery, not even during maximum hyperemia. In case of increased oxygen demand of the myocardium (exercise, stress), the arteriolar sphincters can dilate, and blood flow can increase accordingly, even if perfusion pressure remains unchanged. The mechanism by which sphincters can dilate or contract is dependent, on one hand, on mechanical factors (eg, shear stress) and, on the other hand, on humoral factors (eg, bradykinin, acetylcholine, nitric oxide). If, at exercise, arterial blood pressure further increases (and thereby also the perfusion pressure across the coronary circulation), coronary blood flow will be able to increase further and will always remain sufficient to match the increased oxygen demand and metabolic needs of the myocardium.
The coronary autoregulation also keeps coronary flow constant despite changes in blood pressure. If, for whatever reason, blood pressure decreases, this is also accompanied by dilatation of coronary sphincters to keep coronary perfusion constant despite low blood pressure. If blood pressure increases inadvertently, contraction of sphincters prevents coronary blood flow from increasing inadvertently. In this way, coronary blood flow remains constant between mean aortic pressure from 50 to 130 mm Hg. At average blood pressures of greater than 130 mm Hg, sphincters are maximally contracted, and the further increase of blood pressure will increase coronary perfusion.
Below a mean arterial pressure of 50 mm Hg, the sphincters are maximally dilated, and a further decrease of blood pressure will lead to a pathologic decrease of coronary perfusion. As mentioned, the phenomenon whereby coronary perfusion can be maintained constant over a large range of perfusion pressures is called autoregulation of coronary blood flow.4
Under baseline circumstances, blood flow in the left coronary artery occurs mainly during diastole. In systole, the myocardium compresses its own vasculature, and there is almost no forward blood flow in the left coronary artery. This means that blood flow in the coronary arteries is antiphasic compared to other parts of the human circulation, where blood flow mainly occurs during systole.
During maximum hyperemia of the myocardium (as present during maximum exercise but also after administration of specific vasodilatory drugs), diastolic blood flow in the coronary artery further increases, but also a systolic component in coronary blood flow occurs consisting of 15% to 25% of total coronary blood flow (Fig. 6-2).
FIGURE 6-2
A and B. Blood flow pattern in a normal left coronary artery at rest and at hyperemia. At rest, systolic blood flow is very low, and flow mainly occurs during diastole. During maximum hyperemia, a systolic component also occurs, whereas diastolic blood flow increases even more. C. Example of reactive hyperemia after 20 seconds of occlusion of the left anterior descending artery of a dog. After relief of the occlusion, a rapid increase in blood flow occurs to 500% of resting blood flow, followed by a decrease when the oxygen debt is solved. D. Reactive hyperemia after intracoronary administration of 10 mg of papaverine in the same dog. E. Phasic and mean coronary blood flow in the left anterior descending artery of a dog after occlusions of 3, 5, 10, 20, and 30 seconds. As the time of occlusion increases, reactive hyperemia is more pronounced up to an occlusion time of approximately 20 seconds. With longer occlusions, the peak hyperemia no longer increases, but it takes longer before flow returns to baseline. (From Pijls NHJ. Coronaire fysiologie en myocardischemie. In Van der Wall EE, Van de Werf F, Zijlstra F. Cardiologie. Houten, the Netherlands: Bohn Stafleu van Loghum, 2008, with permission of Springer.22)

The blood flow pattern in the right coronary artery is more variable and more equally distributed across systole and diastole. In case of a dominant right coronary artery, it can look like a flow pattern in the left coronary artery. Normal blood flow patterns at rest and hyperemia are indicated in Figure 6-2.
If, in an experimental model (eg, a dog or a pig), the coronary artery is occluded during some time, myocardial ischemia will occur, and after release of the occlusion, a temporary increase in flow to the myocardium will occur. This is called reactive hyperemia (see Fig. 6-2). The longer the occlusion, the more pronounced and longer is the reactive hyperemia. Reactive hyperemia is maximal after occlusions of at least 20 seconds. If the occlusion lasts longer, the peak of hyperemia will not further increase but recovery of flow to baseline will take longer. Obviously, after occlusions of 20 seconds or more, the arteriolar sphincters are completely dilated. Maximum coronary hyperemia can not only be obtained by temporary occlusion of the coronary artery, but also pharmacologically by administration of specific drugs such as intracoronary papaverine or adenosine or intravenous adenosine, adenosine triphosphate, or regadenoson.5,6
The level to which coronary or myocardial blood flow can increase is indicated by CFR. The absolute CFR is defined as the ratio between hyperemic and baseline blood flow (see Fig. 6-2; Fig. 6-3).7
FIGURE 6-3
Definition and characteristics of coronary flow reserve (CFR) and fractional flow reserve (FFR). In both panels, the relationship between coronary perfusion pressure and coronary blood flow is indicated. Between an average perfusion pressure of 50 and 130 mm Hg, coronary perfusion remains constant at rest (autoregulation, horizontal lines). At maximum exercise or after administration of a vasodilatory stimulus, the arteriolar sphincters in the coronary artery are fully dilated, and a linear relation exist between perfusion pressure and blood flow (oblique lines). In case of a coronary stenosis, the slope of the oblique line will decrease. CFR is defined as maximum blood flow divided by blood flow at rest. Maximum blood flow is dependent on blood pressure, and blood flow at rest varies with heart rate and contractility. Consequently, CFR can vary over a large range for 1 specific coronary stenosis. FFR is defined as maximum blood flow in the presence of a stenosis divided by maximum blood flow in the hypothetical case where no stenosis would be present at all. This ratio is independent of changes in blood pressure, heart rate, and contractility, and under normal circumstances, FFR always has the same reference value (ie, 1.0). An FFR below 0.75 to 0.80 generally corresponds with inducible myocardial ischemia of the territory supplied by that particular coronary artery. In such cases, the stenosis is considered hemodynamically significant, and revascularization is most likely indicated.

As indicated in Figure 6-3 and as is clear from theoretical considerations, CFR correlates with blood pressure and is higher with higher blood pressure. CFR is also dependent on age and decreases with aging. In healthy young people, normal CFR is 5 to 6, but in healthy octogenarians, CFR is often hardly 1.5.
CFR is a useful theoretical parameter to understand the coronary circulation but less suitable in clinical practice to quantify the hemodynamic significance of a coronary stenosis because of its dependency on blood pressure, age, and a number of other factors. In the practice of the interventional laboratory, apart from the lack of a normal reference value, probably the most important limitation is that to determine CFR reliably, one has to rely on resting blood flow. In the catheterization laboratory, in a patient laying on the table and undergoing an intervention, it is hard to be sure that true resting blood flow is present. As a result of these limitations of CFR in clinical studies, the threshold of CFR below which coronary ischemia is inducible varies in different studies from 1.6 to 3.3. This means that the same CFR value of, for example, 2.5 can be completely normal in one person but severely decreased in another person. Consequently, it is difficult to use CFR for decision making with respect to revascularization.
To overcome these shortcomings of CFR for clinical decision making, the concept of fractional flow reserve (FFR) has been developed.8 FFR is defined as maximum achievable blood flow in a stenotic coronary artery compared to maximum blood flow in that same coronary artery in the hypothetical case that the vessel would be normal. As will be explained later, FFR can be determined in the catheterization laboratory by coronary pressure measurement and is useful to characterize the hemodynamic significance of a coronary stenosis and to decide upon sense or nonsense of mechanical revascularization (stenting of bypass surgery). The concept of FFR is illustrated in Figure 6-3 and Figure 6-4 and discussed further later in this chapter.
FIGURE 6-4
Definition of fractional flow reserve (FFR). On the left side, the epicardial coronary artery and the myocardial vascular bed are depicted. In the upper part, no stenosis is present, and normal perfusion pressure during hyperemia is 100 mm Hg. In the lower part, a stenosis is present, and distal coronary pressure at hyperemia has decreased to 70 mm Hg. Therefore, the perfusion pressure has decreased to 70 mm Hg only, whereas it should normally be 100 mm Hg. Because of the linear relationship between hyperemic perfusion pressure and hyperemic blood flow (right part of the figure), this means that the maximum myocardial perfusion in the presence of a stenosis has decreased to only 70% of what would be its normal value. In fact, a ratio of hyperemic blood flows is expressed as a ratio of perfusion pressures, and FFR is the hyperemic distal perfusion pressure (Pd) in the presence of the stenosis divided by the perfusion pressure in the normal case, indicated by Pa. FFR indicates the fraction of normal maximum blood flow that is still achievable in the presence of a stenosis. An FFR of 0.7 means that, as a consequence of the epicardial coronary stenosis, maximum blood flow to the supplied myocardial territory has decreased to 70% of the value it should be in a normal case. Therefore, FFR is a specific measure of the functional severity of a coronary stenosis.

The early phase of atherosclerotic coronary disease is endothelial dysfunction. This is invisible by any imaging method but can be demonstrated by functional testing (Fig. 6-5). In reaction to a number of humoral or mechanical stimuli (eg, increased shear stress), endothelial cells produce nitric oxide (NO), leading to relaxation of underlying smooth muscle cells and vasodilation with increase of blood flow in the coronary artery. The earliest stage of (coronary) atherosclerosis is endothelial dysfunction. This can be demonstrated by intracoronary acetylcholine administration. In the presence of healthy endothelium, acetylcholine also increases NO production and, thereby, coronary blood flow. Acetylcholine also has a direct vasoconstrictive effect on the underlying smooth muscle cells, but in healthy persons, this effect is weaker than the vasodilatory NO-stimulating effect. If the endothelium is diseased, the direct vasoconstrictive effect predominates, and so-called paradoxical vasoconstriction occurs. This phenomenon can be used in the interventional laboratory to demonstrate endothelial dysfunction. Such a test is performed by administrating subselectively intracoronary acetylcholine under controlled conditions but is not trivial and not without risk, and therefore, it should only be applied in specialized catheterization laboratories.
FIGURE 6-5
Abnormal endothelial function. The earliest stage of atherosclerosis is endothelial dysfunction, often present long before abnormalities on the angiogram can be seen. In the left panel, an apparently normal coronary angiogram is seen in a young male with many risk factors and a positive exercise test. To detect abnormal endothelial function, acetylcholine is injected into the coronary artery, resulting in severe and varying vasoconstriction in the different branches of the circumflex system. In the presence of normal endothelium, no such narrowing should be seen. In the right panel, nitroglycerin has been administered to relieve the acetylcholine effects.
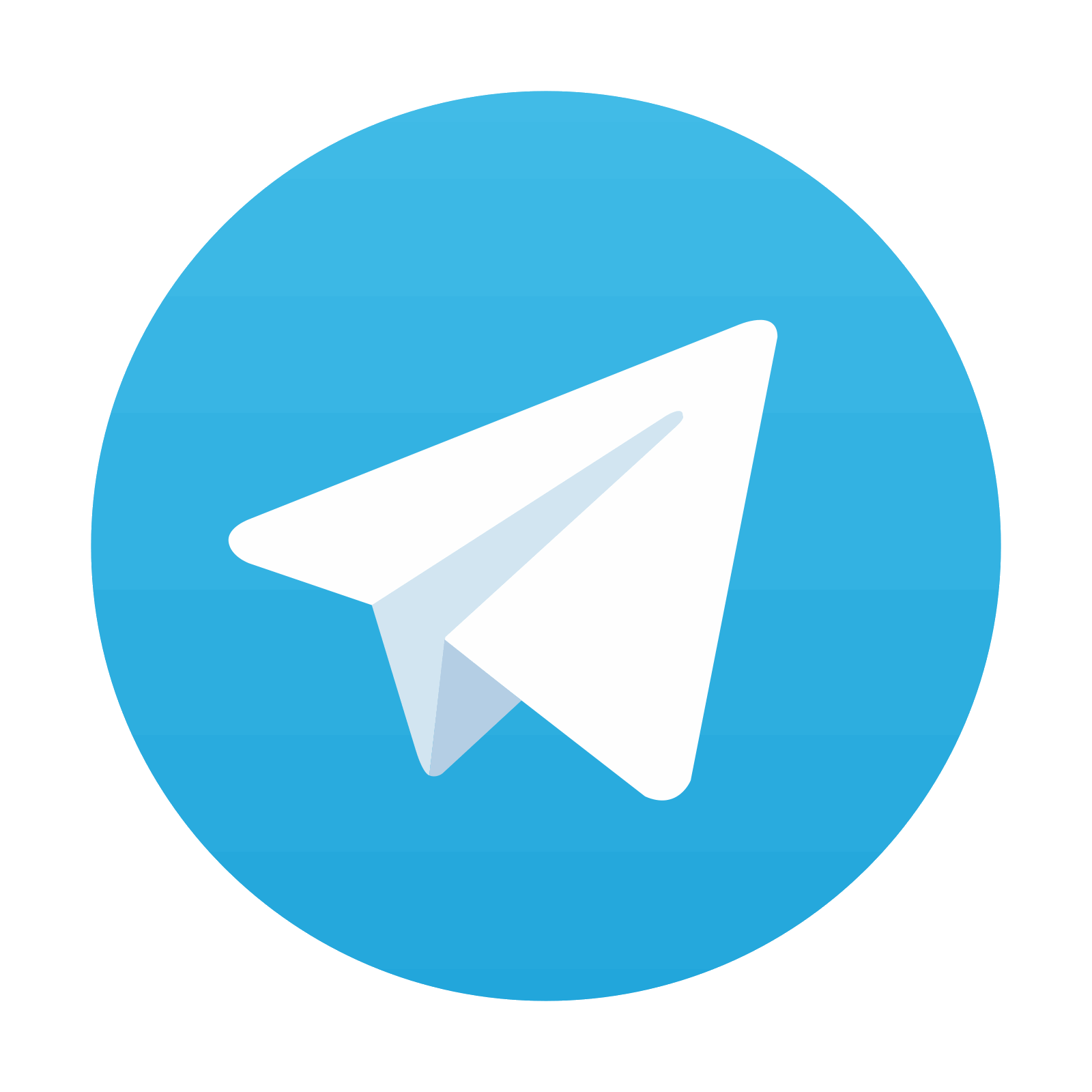
Stay updated, free articles. Join our Telegram channel
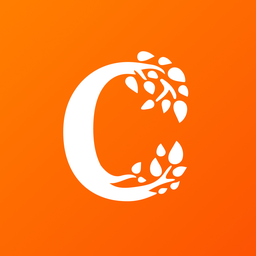
Full access? Get Clinical Tree
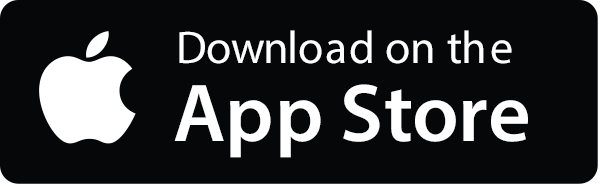
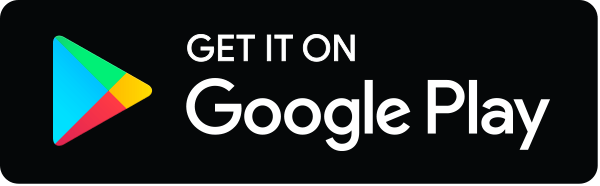
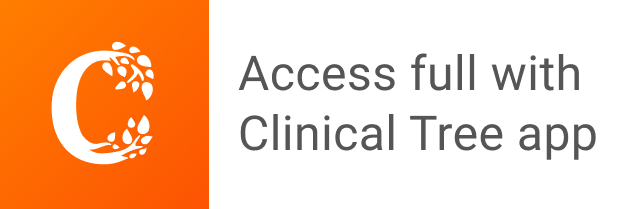