Fig. 17.1
Structure of a cardiac sarcomere. Top: electron microscopy of a cardiomyocyte sarcomere (Photograph Sh. Mahmoodzadeh 2014, with permission). Bottom: schematic interpretation (from: Chapter 6 “Kontraktionsmechanismen”, Linke and Pfitzer; in: “Physiologie des Menschen” 2011; Schmidt, Robert F., Lang, Florian, Heckmann, Manfred (eds); Springer-Verlag, with kind permission from Springer Science + Business Media). Abbreviations: A-band anisotropic band, I-band isotropic band, H-zone “helle Zone” (bright zone), M-band middle band
Sarcomeres consist mainly of longitudinally arranged thin (actin) filaments (polymerized G-actin molecules, around 1 μm in length, 50 nm thick), thick (myosin) filaments (polymerized myosin molecules, around 1.6 μm in length, 100 nm thick), and filaments made of titin (Fig. 17.1). Actin filaments are anchored at the Z-lines, and myosin filaments are centered within the sarcomeres, vertically interconnected by the central M-line. Titin (also called connectin) is a giant elastic protein with spring-like elements in the I-band region, roughly 3900 kDa and 1 μm in length, which directly connects Z- with M-lines of the sarcomere. The actin filament mainly is associated with filamentous tropomyosin as well as troponin complexes. The Z-line with its characteristic zigzag structure (Fig. 17.1) is predominantly composed of globular α-actinin but contains many additional structural and regulatory proteins (e.g., CapZ, desmin, myotilin, telethonin). Costameres are protein assemblies that align the Z-lines of subsarcolemmal myofibrils with the dystrophin-glycoprotein complex (DGC) at the sarcolemma via cytoskeletal actin filaments. Thus, costameres couple force generation of myofibrils with the sarcolemma in striated muscle. The DGC consists of a cytoplasmic syntrophin complex, a transmembrane sarcoglycan complex, and a transmembrane/extracellular dystroglycan complex, which binds to the extracellular matrix protein laminin. The cytoplasmic rod-shaped huge protein dystrophin anchors cytoskeletal actin filaments to the DGC complex.
The structure maintaining the thick filament and titin arrays is the M-line located in the middle of the A-band. The main proteins forming the M-line are muscle-specific creatine kinase, myomesin 2 (M-protein), and myomesin 1.
17.3 The Myosin Filament
According to the sequence of their motor domain, myosins recently were classified into 35 classes [4]. Those myosins which form dimers and assemble into thick filaments are called conventional or type II myosins and were first roughly prepared and named by W. Kühne in 1863 [5].
Type II myosins (constituting around 35 % of the total skeletal muscle protein) are the motor proteins of all muscle types. They are composed of two heavy chains (MYH). Each MYH associates with two different light chains (MLC), the essential (ELC) and regulatory (RLC) light chains.
The MYH (e.g., chicken skeletal MYH = 1938aa, ≈200 kDa) can be proteolytically cleaved into a C-terminal 150 nm alpha-helical rod domain and a pear-shaped ≈ 20 nm N-terminal head domain with associated MLC, called subfragment 1 or S-1. Proteolytic cleavage of the myosin rod domain yields subfragment 2 (S-2) and light meromyosin (LMM). They contain multiple heptad-repeat sequences, allowing dimerization to form a coiled-coil superstructure. S-1 and S-2 together are termed heavy meromyosin (HMM). Limited proteolysis of S-1 produces (from N- to C-terminus) a 25 K, a 50 K (with a large cleft and the actin-binding sites), and a 20 K domain, the converter domain, and the α-helical lever arm. The ATPase binding site forms a cleft at the 25 K/50 K interface. The lever arm contains two IQ motifs in tandem, namely, IQ1 for ELC binding and IQ2 for RLC binding [6]. Full-length ELC is designated as alkali 1 (A1), while alternatively spliced ELC forms with the N-terminal 46aa deleted are termed alkali 2 (A2). The C-terminus of A1 with its four EF-hand domains binds to the myosin lever arm while its antenna-like N-terminus interacts with the thin filament.
The coiled-coil rod domains of myosin associate to form end-polar (striated muscles) or side-polar (smooth muscle) myosin filaments. Each end-polar myosin filament (≈1.6 μm in length, 100 nm in diameter; thick filament) contains about 300 myosin molecules. The myosin heads of end-polar thick filament project in a helical array with a periodicity of 42.9 nm and an axial interval of 14.3 nm – except for a 0.15 μm bare zone, where there are only overlapping myosin rods. The myosin heads bind as “cross-bridges” (XB) to specific sites on the actin filament for contraction generation. The myosin filament is associated with myosin-binding protein C (C-protein) at regular intervals.
17.4 The Actin Filament
Actin (which comprises about 19 % of total skeletal muscle protein) is a globular protein (G-actin) with a 5.5 nm diameter and molecular weight of 42 kDa. G-actin can be divided into four subdomains with a central ATP bound. G-actin molecules polymerize under physiological conditions to form a helical filamentous structure (F-actin) with a length of 1 μm and diameter of 50 nm (thin filament), each actin with a central ADP bound. In particular, subdomain 1 which contains both the N- and C-termini of actin is located at the periphery of the thin filament and available for myosin interactions. F-actin appears by electron microscopy as two twisting strands of globular subunits. The axial separation of the crossover points is about 35 nm; thus every 7th actin has the same orientation.
F-actin is anchored to the Z-line by its plus end (i.e., the filament site of preferential growth) and binds to the capping protein tropomodulin at its minus end. Myosin-S1 forms arrowhead complexes with actin filaments which point to the minus end (i.e., toward the center of the sarcomere). Hence, type II myosin is termed a minus-end-directed motor. The final thin filament contains about 200 actin monomers associated with regulatory troponin-tropomyosin complexes (ca. 30 tropomyosin molecules and ca. 30 troponin complexes) regularly spaced along the thin filament.
Tropomyosin (Tm) consists of two α-helical polypeptide chains, each of ca. 35 kDa, forming a ≈42-nm-long coiled coil. Tm molecules aggregate end to end to form continuous strands running along the actin filament. Interactions between tropomyosin and actin are electrostatic.
The troponin (Tn) complex consists of three components, TnI, TnT, and TnC, with a total molecular weight of ≈80 kDa. Tn complexes are located every 35 nm on F-actin (i.e., every seven actins along the long pitch helix), probably at the sites of overlap between neighboring tropomyosin molecules. TnT (≈36 kDa) is a rod-like protein (18.5 nm long) which binds with its C-terminal half to both TnI and TnC and with its N-terminal half to both Tm and actin. TnI (≈24 kDa) holds the Tn complex together by binding to both TnC and actin. Cardiac TnI has a ≈30aa N-terminal extension with several phosphorylation sites. TnC (≈18 kDa) is the Ca2+ sensor of the contractile apparatus. It has a dumbbell-like shape with an N- and a C-terminal lobe. Binding of Ca2+ to TnC at the low-affinity sites at the N-lobe (two sites in skeletal, one site in cardiac TnC) represents the physiological trigger regulating contraction. The C-lobe of TnC (containing two high-affinity Ca2+/Mg2+ binding sites) interacts with TnI and TnT.
In addition to the regulatory Tn-Tm complexes, F-actin associates with nebulin filaments (skeletal muscle ~700 kDa) or its smaller cardiac homologue nebulette (~100 kDa). Nebulin binds to actin and Tn-Tm complexes and is anchored to the C-terminus of the Z-disk, where it binds via its N-terminus to tropomodulin. Nebulin and nebulette act as molecular rulers that determine thin filament length and may also modulate actomyosin interactions.
17.5 Cardiomyocyte Contraction
Cardiomyocytes have characteristic passive as well as active properties. Passive elasticity, i.e., tension generated upon stretch during rest, mainly depends on the elastic titin filaments. Furthermore, compression of titin spring elements during sarcomere shortening may provide the restoring force that sets the sarcomere length to resting levels when activation ceases.
An activated cardiomyocyte contracts, i.e., generates force and shortens with a load. Force generation without shortening is termed isometric and shortening at constant load/force generation, isotonic. The energy for muscle contraction comes from the hydrolysis of ATP. Hydrolyzed ATP can rapidly be resynthesized by different metabolic processes. ATP could effectively be resynthesized by transfer of Pi from phosphocreatine to ADP through the activity of creatine kinase (Lohmann reaction). Adenylate kinase (or myokinase) produces ATP by conversion of two ADP molecules to one ATP and one AMP molecule, which is removed by AMP deaminase to form inosine monophosphate and NH4. In the heart, ATP is mainly synthesized through aerobic metabolism, i.e., conversion of glucose, fatty acids, and lactate to acetyl CoA and subsequent oxidation to CO2 and H2O in the mitochondria (Krebs cycle, electron transport chain). Hence oxygen consumption of the heart (around 0.1 ml/g/min) is high, accounting for approximately 10 % of the total oxygen consumption of the body.
Mechanochemical energy transformation is accomplished by cyclic interaction of the cross-bridges (XBs) with the thin filament. During this process, the XBs bind independently, generate force through a conformational change (the power stroke), and detach from the thick filaments (the cross-bridge theory; [7]). Recent X-ray crystallographic 3-D analysis of myosin-S1 fixed at different states of the XB cycle revealed the domain movements. According to a recently modified model [8] (Fig. 17.2), ATP binds to the ATP-binding cleft of the myosin XB (M), which causes the rapid detachment of the XB from the actin (A) filament (A + M-ATP). ATP is hydrolyzed, forming the M-ADP-Pi state (pre-power-stroke state, lever arm up, 50 K-cleft open). The M-ADP-Pi state weakly attaches to actin through ionic interactions (A-M-ADP-Pi). Releasing Pi then forms a strong stereospecific actin-binding state required for the power stroke of the XB (A-M-ADP). The power stroke is executed by movement of the myosin lever arm by ≈10 nm (lever arm down, 50 K-cleft closed). Detachment of the force-generating XB from the actin filament is achieved by the release of ADP and binding of ATP and restoration of the lever arm of the XB to its non-force-generating position (recovery stroke). In the absence of ATP, the nucleotide-free XBs remain strongly bound to the actin filament producing a rigor mortis state (A-M; lever arm down, 50 K-cleft closed; the swinging lever arm model [9]). Crystal structures have also shown that a small conformational change in the ATP-binding domain of the XB (movement of 0.5 nm) is amplified into the ≈10 nm movement of the lever arm.
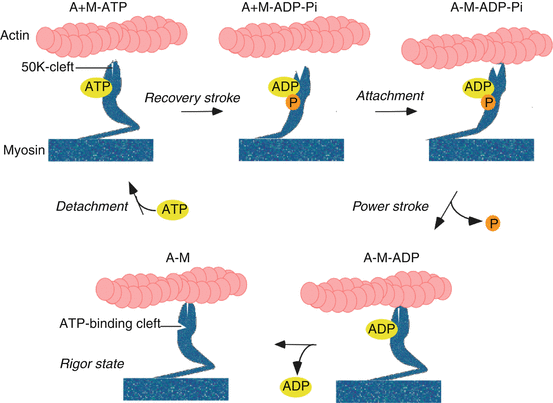
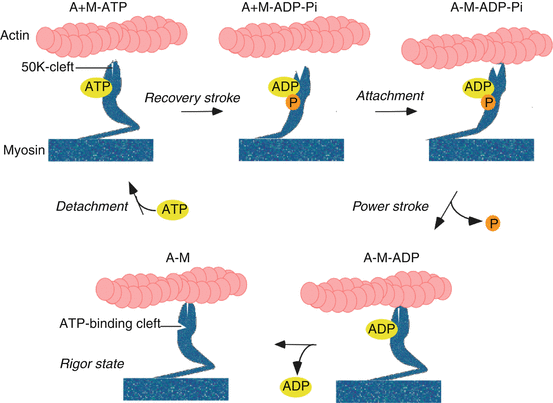
Fig. 17.2
Simplified scheme of chemomechanical coupling. A−M: the myosin cross-bridge is bound in a nucleotide-free force-generating state to actin (rigor position; lever arm “down,” 50 K-cleft closed). A+M-ATP: ATP binds to myosin which detaches from actin. A+M−ADP−Pi: ATP is quickly hydrolyzed moving the lever arm “up,” 50 K-cleft open (recovery stroke). A−M−ADP−Pi: myosin attaches to actin in a non-force-generating pre-power-stroke state (lever arm up, 50 K-cleft open); A−M−ADP: upon release of P i , myosin is shifting into its force-generating state (power stroke), with the lever arm “down,” 50 K-cleft closed. A−M: Release of ADP forms the transient rigor state; rapid binding of ATP to the active site of the myosin cross-bridge then causes the rapid detachment of the A−M into the A+M−ATP state. Abbreviations: A actin, M myosin, P i inorganic phosphate, ATP adenosine triphosphate, ADP adenosine diphosphate
17.5.1 Isometric Contraction
Isometric force generation of a muscle cell (F 0) during steady-state contraction depends on the number of XBs in the force-generating state and the force a XB can exert (F′, ≈6 pN). Since XBs independently interact with the thin filament during muscle activation, only a fraction “n” of the total amount of actively cycling XBs (n tot) generates force at any one time. “n” therefore depends on the XB-kinetics, i.e., the rates of transitions of XBs from force- into the non-force-generating states (detachment rate; g app) and the rate of transitions of XBs from non-force- into force-generating states (attachment rate; f app), namely:
There is a bell-shaped dependency of force generation on the muscle/sarcomere length. The optimal force is elicited at resting sarcomere length, i.e., around 2 μm, while smaller or larger sarcomere lengths gradually decrease force generation. The level of thick and thin filaments overlaps, and the resulting different amounts of force-generating XBs provide the structural basis for this observation [11]. The heart is working on the ascending limb of the length-tension curve. An increased filling volume of the heart, which sets the sarcomeres into a more optimal filament overlap zone, could therefore be acutely compensated by an increased force generation and stroke volume (Frank-Starling mechanism). In addition, Ca2+ sensitivity of the myofilaments increases upon stretching the sarcomeres, supporting the increased force and ejection volume after increased diastolic filling.
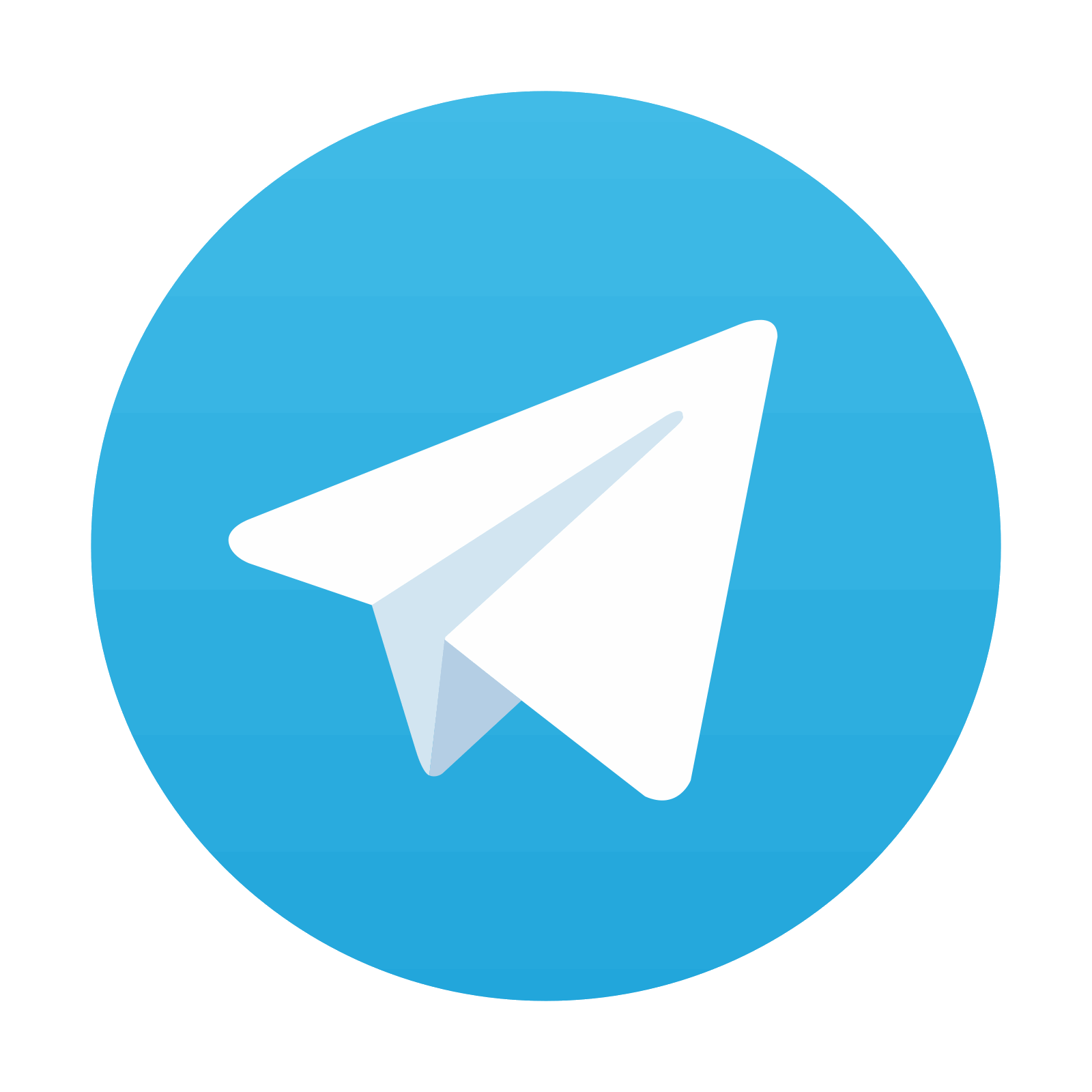
Stay updated, free articles. Join our Telegram channel
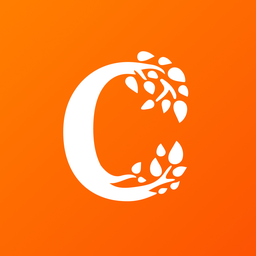
Full access? Get Clinical Tree
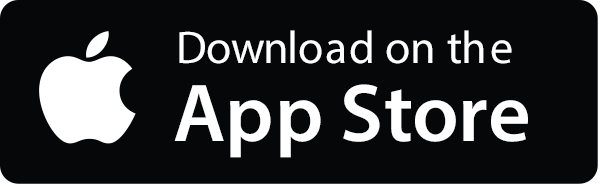
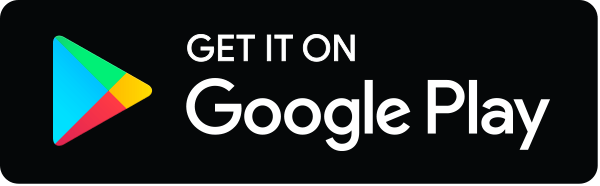