For additional ancillary materials related to this chapter. please visit thePoint.
In this chapter you will learn:
1 | how the electrical current in the heart is generated
2 | how this current is propagated through the four chambers of the heart
3 | that the movement of electricity through the heart produces predictable wave patterns on the EKG
4 | how the EKG machine detects and records these waves
5 | that the EKG looks at the heart from 12 different perspectives, providing a remarkable three-dimensional electrical map of the heart
6 | that you are now able to recognize and understand all the lines and waves on the 12-lead EKG
7 | that trusting the EKG machine to interpret your patient’s EKG is nothing but an invitation to trouble!
Electricity and the Heart
Electricity, an innate biologic electricity, is what makes the heart go. The EKG is nothing more than a recording of the heart’s electrical activity, and it is through perturbations in the normal electrical patterns that we are able to diagnose many different cardiac and noncardiac disorders.
All You Need to Know About Cellular Electrophysiology in a Few Brief Paragraphs
Cardiac cells, in their resting state, are electrically polarized; that is, their insides are negatively charged with respect to their outsides. This electrical polarity is maintained by membrane pumps that ensure the appropriate distribution of ions (primarily potassium, sodium, chloride, and calcium) necessary to keep the insides of these cells relatively electronegative. These ions pass into and out of the cell through special ion channels in the cell membrane.

Sometimes lethal disturbances in the conduction of electricity through the heart occur because of an inherited disorder of these transmembrane ion channels. Fortunately, these so-called channelopathies are quite rare. Many different genetic mutations affecting the cardiac ion channels have been identified, and more are being discovered every year.
Cardiac cells can lose their internal negativity in a process called depolarization. Depolarization is the fundamental electrical event of the heart. In some cells, known as pacemaker cells, it occurs spontaneously. In others, it is initiated by the arrival of an electrical impulse that causes positively charged ions to cross the cell membrane.
Depolarization is propagated from cell to cell, producing a wave of depolarization that can be transmitted across the entire heart. This wave of depolarization represents a flow of electricity, an electrical current, that can be detected by electrodes placed on the surface of the body.
After depolarization is complete, the cardiac cells restore their resting polarity through a process called repolarization. Repolarization is accomplished by the membrane pumps, which reverse the flow of ions. This process can also be detected by recording electrodes.
All of the different waves that we see on an EKG are manifestations of these two processes: depolarization and repolarization.

The Cells of the Heart
From the standpoint of the electrocardiographer, the heart consists of three types of cells:
- Pacemaker cells—under normal circumstances, the electrical power source of the heart
- Electrical conducting cells—the hard wiring of the heart
- Myocardial cells—the contractile machinery of the heart

Pacemaker Cells
Pacemaker cells are small cells approximately 5 to 10 μm long, about the same as the width of a single strand of a spider’s web. These cells are able to depolarize spontaneously over and over again. The rate of depolarization is determined by the innate electrical characteristics of the cell and by external neurohormonal input. Each spontaneous depolarization serves as the source of a wave of depolarization that initiates one complete cycle of cardiac contraction and relaxation.
If we record one electrical cycle of depolarization and repolarization from a single cell, we obtain an electrical tracing called an action potential. With each spontaneous depolarization, a new action potential is generated, which in turn stimulates neighboring cells to depolarize and generate their own action potential, and so on and on, until the entire heart has been depolarized.

The action potential of a cardiac pacemaker cell looks a little different than the generic action potential shown here. A pacemaker cell does not have a true resting potential. Its electrical charge drops to a minimal negative potential of approximately −60 mV, which it maintains for just a moment (it does not rest there), and then gradually rises until it reaches the threshold for the sudden depolarization that is an action potential. These events are illustrated on the following tracing.

The dominant pacemaker cells in the heart are located high up in the right atrium. This group of cells is called the sinoatrial (SA) node, or sinus node for short. These cells typically fire at a rate of 60 to 100 times per minute, but the rate can vary tremendously depending upon the activity of the autonomic nervous system (e.g., sympathetic stimulation from catecholamines, such as epinephrine and norepinephrine, accelerates the sinus node, whereas vagal stimulation slows it) and the demands of the body for increased cardiac output (exercise raises the heart rate, whereas a restful afternoon nap lowers it).
Pacemaker cells are really good at what they do. They will continue firing in a donor heart even after it has been harvested for transplant and before it has been connected to the new recipient. The transplanted heart, devoid of normal vagal stimulation (the nerves are cut when the new heart is implanted), beats at an average rate of 100 beats per minute (bpm).

Actually, every cell in the heart has the ability to behave like a pacemaker cell. This so-called automatic ability is normally suppressed unless the dominant cells of the sinus node fail or if something in the internal or external environment of a cell (sympathetic stimulation, cardiac disease, etc.) stimulates its automatic behavior. This topic assumes greater importance later on and is discussed under Ectopic Rhythms in Chapter 3.
Electrical Conducting Cells
Electrical conducting cells are long, thin cells. Like the wires of an electrical circuit, these cells carry current rapidly and efficiently to distant regions of the heart. They are, in effect, the electrical highway of the heart.
The electrical conducting cells of the ventricles form distinct electrical pathways. The ventricular conducting fibers constitute what is called the Purkinje system.
The conducting pathways in the atria have more anatomic variability; prominent among these are fibers at the top of the intra-atrial septum in a region called Bachmann’s bundle that allow for rapid activation of the left atrium from the right.

Myocardial Cells
The myocardial cells constitute by far the largest part of the heart tissue. They are responsible for the heavy labor of repeatedly contracting and relaxing, thereby delivering blood to the rest of the body. These cells are about 50 to 100 μm in length and contain an abundance of the contractile proteins actin and myosin.
When a wave of depolarization reaches a myocardial cell, calcium is released within the cell, causing the cell to contract. This process, in which calcium plays the key intermediary role, is called excitation–contraction coupling.

Myocardial cells can transmit an electrical current just like electrical conducting cells, but they do so far less efficiently. Thus, a wave of depolarization, upon reaching the myocardial cells, will spread slowly across the entire myocardium.
Time and Voltage
The waves that appear on an EKG primarily reflect the electrical activity of the myocardial cells, which make up the vast bulk of the heart. Pacemaker activity and transmission by the conducting system are generally not seen on the EKG; these events simply do not generate sufficient voltage to be recorded by surface electrodes.
The waves produced by myocardial depolarization and repolarization are recorded on EKG paper and, like any wave, have three chief characteristics:
- Duration, measured in fractions of a second
- Amplitude, measured in millivolts (mV)
- Configuration, a more subjective criterion referring to the shape and appearance of a wave

EKG Paper
EKG paper is a long, continuous roll of graph paper, usually pink (but any color will do), with light and dark lines running vertically and horizontally. The light lines circumscribe small squares of 1 × 1 mm; the dark lines delineate large squares of 5 × 5 mm.
The horizontal axis measures time. The distance across one small square represents 0.04 seconds. The distance across one large square is five times greater, or 0.2 seconds.
The vertical axis measures voltage. The distance along one small square represents 0.1 mV, and along one large square, 0.5 mV.
You will need to memorize these numbers at some point, so you might as well do it now.

P Waves, QRS Complexes, T Waves, and Some Straight Lines
Let’s follow one cycle of cardiac contraction (systole) and relaxation (diastole), focusing on the electrical events that produce the basic waves and lines of the standard EKG.
Atrial Depolarization
The sinus node fires spontaneously (an event not visible on the EKG), and a wave of depolarization begins to spread outward into the atrial myocardium, much as if a pebble were dropped into a calm lake. Depolarization of the atrial myocardial cells results in atrial contraction.

During atrial depolarization and contraction, electrodes placed on the surface of the body record a small burst of electrical activity lasting a fraction of a second. This is the P wave. It is a recording of the spread of depolarization through the atrial myocardium from start to finish.
Because the sinus node is located in the right atrium, the right atrium begins to depolarize before the left atrium and finishes earlier as well. Therefore, the first part of the P wave predominantly represents right atrial depolarization, and the second part left atrial depolarization.
Once atrial depolarization is complete, the EKG again becomes electrically silent.

A Pause Separates Conduction From the Atria to the Ventricles
In healthy hearts, there is an electrical gate at the junction of the atria and the ventricles. The wave of depolarization, having completed its journey through the atria, is prevented from communicating with the ventricles by the heart valves that separate the atria and ventricles. Electrical conduction must be funneled along the interventricular septum, the wall that separates the right and left ventricles. Here, a structure called the atrioventricular (AV) node slows conduction to a crawl. This pause lasts only a fraction of a second.
This physiologic delay in conduction is essential to allow the atria to finish contracting before the ventricles begin to contract. This clever electrical wiring of the heart permits the atria to empty their volume of blood completely into the ventricles before the ventricles contract.
Like the sinus node, the AV node is also under the influence of the autonomic nervous system. Vagal stimulation slows the current even further, prolonging the delay, whereas sympathetic stimulation accelerates the current through the AV node.

Ventricular Depolarization
After about one-tenth of a second, the depolarizing wave escapes the AV node and is swept rapidly down the ventricles along specialized electrical conducting cells.
This ventricular conducting system has a complex anatomy but essentially consists of three parts:
- Bundle of His
- Bundle branches
- Terminal Purkinje fibers

The bundle of His emerges from the AV node and almost immediately divides into right and left bundle branches. The right bundle branch carries the current down the right side of the interventricular septum all the way to the apex of the right ventricle. The left bundle branch is more complicated. It divides into three major fascicles:
- Septal fascicle, which depolarizes the interventricular septum (the wall of muscle separating the right and left ventricles) in a left-to-right direction
- Anterior fascicle, which runs along the anterior wall of the left ventricle
- Posterior fascicle, which sweeps over the posterior wall of the left ventricle
The right bundle branch and the left bundle branch and its fascicles terminate in countless tiny Purkinje fibers, which resemble little twigs coming off the branches of a tree. These fibers deliver the electrical current into the ventricular myocardium.

Ventricular myocardial depolarization causes ventricular contraction. It is marked by a large deflection on the EKG called the QRS complex. The amplitude of the QRS complex is much greater than that of the atrial P wave because the ventricles have so much more muscle mass than the atria. The QRS complex is also more complicated and variable in shape than the P wave, reflecting the greater intricacy of the pathway of ventricular depolarization.

The Parts of the QRS Complex
The QRS complex consists of several distinct waves, each of which has a name. Because the precise configuration of the QRS complex can vary so greatly, a standard format for naming each component has been devised. It may seem a bit arbitrary to you right now, but it actually makes good sense.
- If the first deflection is downward, that part of the QRS complex is called a Q wave.
- The first upward deflection is called an R wave.
- If there is a second upward deflection, it is called R′ (“R-prime”).
- The first downward deflection following an upward deflection is called an S wave. Therefore, if the first wave of the complex is an R wave, the ensuing downward deflection is called an S wave, not a Q wave. A downward deflection can only be called a Q wave if it is the first wave of the complex. Any other downward deflection is called an S wave.
- If the entire configuration consists solely of one downward deflection, the wave is called a QS wave.
Here are several of the most common QRS configurations, with each wave component named.

The earliest part of the QRS complex represents depolarization of the interventricular septum by the septal fascicle of the left bundle branch. The right and left ventricles then depolarize at about the same time, but most of what we see on the EKG represents left ventricular activation because the muscle mass of the left ventricle is about three times that of the right ventricle.

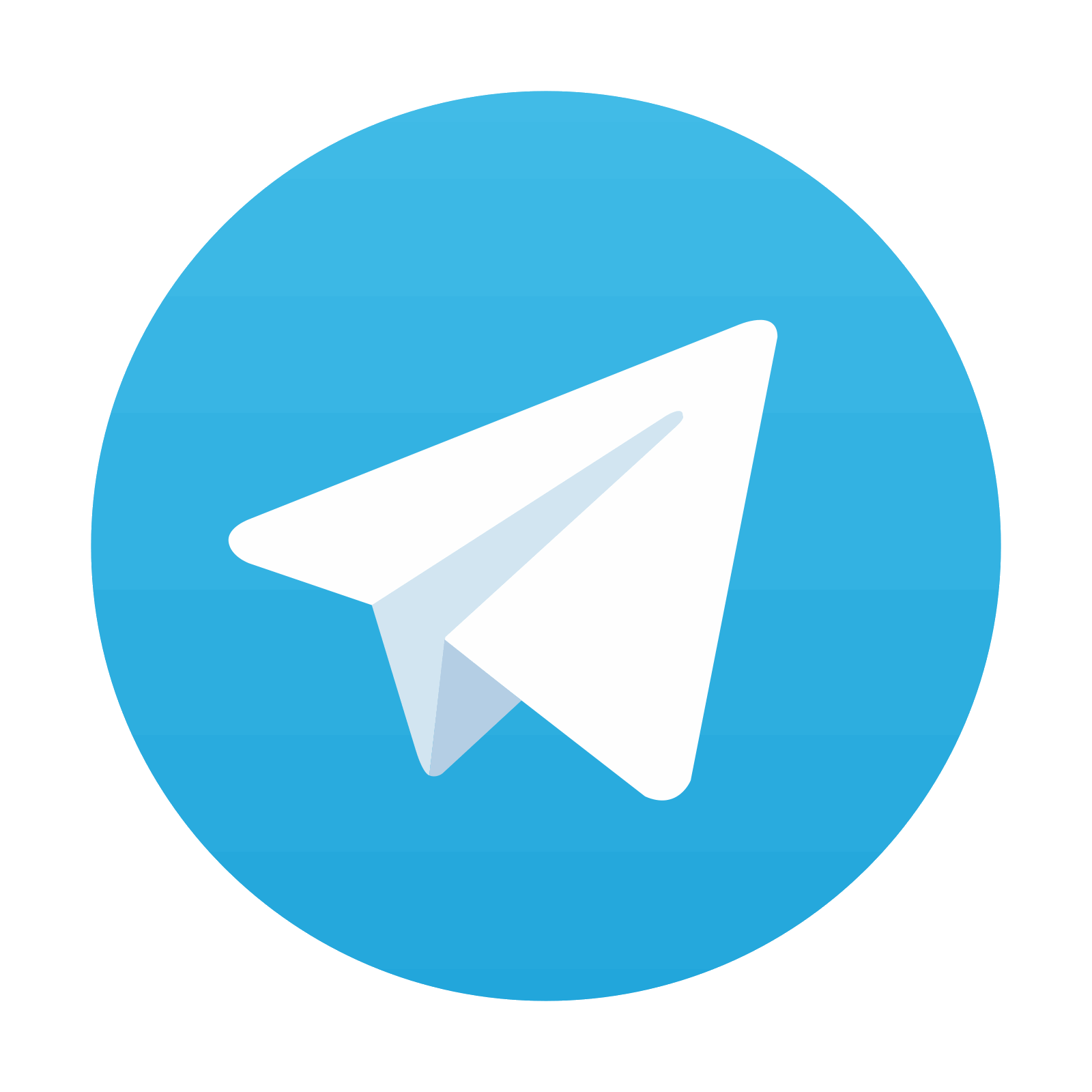
Stay updated, free articles. Join our Telegram channel
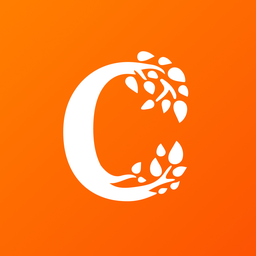
Full access? Get Clinical Tree
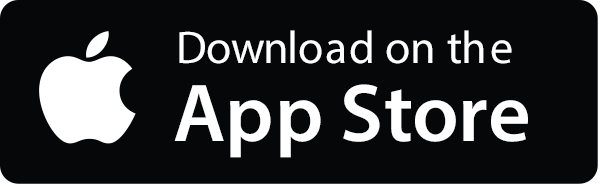
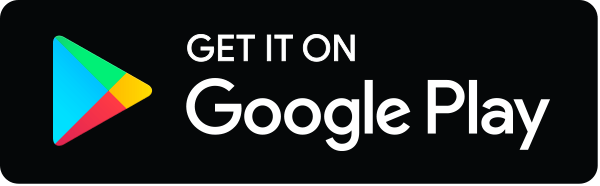
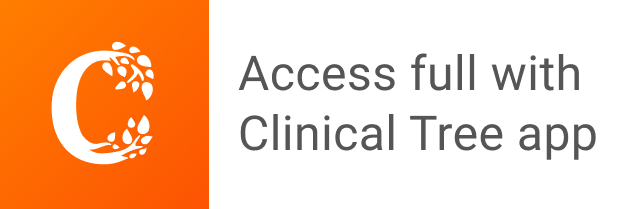