Abstract
Thalassemia is a hereditary blood disorder characterized by reduced hemoglobin production, leading to chronic anemia. A major complication of thalassemia is iron overload, primarily due to regular blood transfusions and increased gastrointestinal iron absorption, which can lead to iron overload cardiomyopathy, a significant cause of morbidity and mortality in thalassemia patients. This review aims to provide an in-depth analysis of the pathophysiological mechanisms underlying iron overload cardiomyopathy in thalassemia, examining how excessive iron accumulation disrupts cardiac function through oxidative stress, cellular damage, and altered calcium homeostasis. Clinical manifestations, including fatigue, arrhythmias, and heart failure, are discussed alongside diagnostic strategies such as echocardiography and cardiac MRI for early detection and monitoring. Management approaches focusing on iron chelation therapy, lifestyle modifications, and advanced interventions like gene therapy are explored. The review also highlights the importance of early diagnosis, regular monitoring, and patient adherence to therapy to prevent the progression of cardiomyopathy. Recent advances in treatment and future research directions, including personalized medicine, and gene editing technologies, are presented. Addressing the challenges in managing iron overload in thalassemia patients is crucial for improving outcomes and enhancing quality of life.
Introduction
Thalassemia is a group of recessively inherited blood disorders characterized by the body’s inability to produce adequate amounts of hemoglobin, leading to chronic anemia and related health complications. , The disease results from mutations in the genes responsible for hemoglobin production, causing the affected individuals to produce either no or very low levels of functional hemoglobin, which is essential for oxygen transport in the blood. Thalassemia can be broadly categorized into two main types: alpha and beta thalassemia. Alpha thalassemia occurs when there is a deletion or mutation in one or more of the four alpha-globin genes, leading to a reduction or absence of alpha-globin chains. Beta thalassemia, on the other hand, is caused by mutations in the beta-globin genes, leading to a lack of beta-globin chain production. , The co-inheritance of beta thalassemia traits and structural hemoglobin variants (e.g., hemoglobin S, C, and E) is common, forming hemoglobin S/β-thalassemia, C/β-thalassemia, and hemoglobin E/β-thalassemia respectively. Overall, these genetic alterations result in imbalanced globin chain synthesis, ineffective erythropoiesis, and chronic hemolysis.
The prevalence of thalassemia varies geographically, with a high concentration of cases in the Mediterranean region, Southeast Asia, the Indian subcontinent, and the Middle East. , It is estimated that approximately 5% of the global population carries thalassemia-related genetic traits, with 1.5% specifically carrying the beta-thalassemia trait. , , The disease presents significant public health challenges, particularly in regions where thalassemia is endemic, due to the need for regular blood transfusions and specialized medical care. These frequent blood transfusions, essential for managing anemia in thalassemia patients, contribute to iron overload, a major complication associated with thalassemia management. Iron overload occurs as the body’s capacity to regulate iron absorption is overwhelmed by the excess iron introduced through transfusions, as well as increased gastrointestinal absorption, leading to accumulation in vital organs such as the heart, liver, and endocrine glands. Addressing iron overload is crucial because the excess iron deposits in tissues can cause severe complications, including liver cirrhosis, diabetes, and most critically, iron overload cardiomyopathy (IOC), which is a leading cause of morbidity and mortality in thalassemia patients. , IOC results from the toxic effects of excess iron on cardiac myocytes, leading to oxidative stress, cellular damage, and heart dysfunction. These complications underscore the importance of regular monitoring and management of iron levels in thalassemia patients to prevent long-term organ damage.
This review aims to provide a comprehensive analysis of the pathophysiological mechanisms, clinical manifestations, and management strategies for IOC in thalassemia. By synthesizing the latest research findings and clinical practices, this review seeks to highlight the importance of early diagnosis, effective treatment, and ongoing research to improve patient outcomes and quality of life in individuals affected by thalassemia. The review also discusses recent advancements in the field, including novel therapeutic approaches and future directions for research, to provide a holistic understanding of the challenges and potential solutions in managing IOC in thalassemia patients.
Pathophysiology of thalassemia
The pathophysiology of thalassemia is rooted in its genetic basis, where mutations in the genes responsible for hemoglobin production lead to the clinical manifestations of the disease. Thalassemia primarily involves mutations in the alpha or beta globin genes ( Table 1 ), which disrupt the normal balance of globin chain synthesis. , In alpha-thalassemia, deletions or mutations in one or more of the four alpha-globin genes reduce the production of alpha-globin chains, while beta-thalassemia results from mutations in the beta-globin genes, leading to insufficient beta-globin chains. These genetic alterations result in imbalanced globin synthesis, which is a hallmark of thalassemia, causing the precipitation of unpaired globin chains, oxidative damage, and ineffective erythropoiesis. ,
Globin Chain Type | Description | Role in Thalassemia | Affected Thalassemia Types |
---|---|---|---|
Alpha (α) Globin | One of the two major types of globin chains that make up hemoglobin. Each hemoglobin molecule contains two alpha chains. | In alpha-thalassemia, mutations or deletions in the alpha-globin genes reduce or eliminate alpha chain production. The absence of alpha chains leads to an excess of beta, gamma, or delta chains, resulting in unstable hemoglobin. | Alpha-thalassemia |
Beta (β) Globin | The other major type of globin chain, paired with alpha chains in hemoglobin. Each hemoglobin molecule contains two beta chains. | In beta-thalassemia, mutations in the beta-globin gene reduce or eliminate beta chain production. A lack of beta chains leads to an excess of alpha chains, causing precipitation and ineffective erythropoiesis. | Beta-thalassemia |
Gamma (γ) Globin | Found in fetal hemoglobin (HbF), which predominates in the fetus and newborns. HbF is composed of two alpha and two gamma chains. | Increased gamma globin production is a compensatory response to reduced beta-globin in beta-thalassemia patients. HbF has a higher affinity for oxygen and can help ameliorate anemia in beta-thalassemia. | Beta-thalassemia |
Delta (δ) Globin | Present in a minor adult hemoglobin (HbA2), which consists of two alpha and two delta chains. Normally makes up a small percentage of adult hemoglobin (around 2-3.5%). | HbA2 levels are elevated in beta-thalassemia as a compensatory mechanism due to reduced beta-globin production. Elevated HbA2 is a diagnostic marker for beta-thalassemia. | Beta-thalassemia |
Zeta (ζ) Globin | An embryonic globin chain found in early development. Part of embryonic hemoglobin (Hb Gower-1). | Mutations in zeta globin genes are rare and not typically involved in thalassemia. | Not directly involved in common thalassemias |
Epsilon (ε) Globin | Another embryonic globin chain involved in early hemoglobin production. Found in embryonic hemoglobin (Hb Gower-2). | Similar to zeta, mutations are rare and not commonly associated with thalassemia. | Not directly involved in common thalassemias |
Thalassemia is classified into three clinical forms: thalassemia major, thalassemia intermedia, and thalassemia minor (or thalassemia trait), each differing in severity based on the genetic mutations involved and their impact on hemoglobin production ( Table 2 ). , Thalassemia major, also known as Cooley’s anemia, is the most severe form and is typically caused by homozygous or compound heterozygous mutations that completely abolish beta-globin chain production. This results in a severe imbalance between alpha and beta chains, leading to excessive unpaired alpha chains that precipitate within red blood cells. The precipitation causes ineffective erythropoiesis and severe anemia requiring regular blood transfusions to maintain adequate hemoglobin levels. In thalassemia major, hemoglobin electrophoresis typically reveals a marked reduction or absence of hemoglobin A (HbA), with elevated levels of hemoglobin F (HbF) and sometimes hemoglobin A2 (HbA2). , ,
Characteristics | Thalassemia Major | Thalassemia Intermedia | Thalassemia Minor (Trait) | Normal Values |
---|---|---|---|---|
Genetic Basis | Homozygous or compound heterozygous mutations | Mild homozygous or compound heterozygous mutations | Heterozygous mutation | No mutation |
Hemoglobin Level | Severely low (<7 g/dL) | Moderately low (7-10 g/dL) | Mildly low or near normal (10-12 g/dL) | Normal (12-16 g/dL for females, 13-17 g/dL for males) |
Clinical Presentation | Severe anemia, failure to thrive, jaundice | Mild to moderate anemia, occasional jaundice | Asymptomatic or mild anemia, often detected incidentally | No symptoms |
Blood Transfusion Requirement | Regular (every 2-4 weeks) | Occasional to frequent | Rarely required | None |
Iron Overload | High risk due to frequent transfusions | Moderate risk | Low risk | No risk |
Hb Electrophoresis Findings | HbA: Absent or very low | HbA: Present in reduced quantities | HbA: Normal to slightly reduced | HbA: 95-98% |
HbF: Elevated (70-90%) | HbF: Elevated (10-60%) | HbF: Normal or slightly elevated (1-5%) | HbF: <1% | |
HbA2: Normal to elevated (4-10%) | HbA2: Elevated (4-10%) | HbA2: Elevated (3.5-7%) | HbA2: 2-3.5% | |
Management Approach | Regular transfusions, intensive chelation therapy | Occasional transfusions, chelation as needed | Monitoring, no regular transfusions, genetic counselling | No specific management needed |
Life Expectancy | Reduced without treatment, near-normal with optimal care | Near-normal with proper management | Normal | Normal |
Thalassemia intermedia presents with a milder clinical phenotype compared to thalassemia major, with symptoms ranging from mild to moderate anemia, which often does not necessitate regular blood transfusions. This form of thalassemia arises from less severe genetic mutations that allow for some production of beta-globin chains, leading to a partial imbalance between alpha and beta chains. Patients with thalassemia intermedia usually show variable levels of hemoglobin F and a mild to moderate increase in hemoglobin A2 on electrophoresis, with hemoglobin A still being present but in reduced quantities compared to normal individuals. The variable clinical manifestations of thalassemia intermedia can be attributed to the genetic heterogeneity of the disease, including co-inheritance of alpha-thalassemia or persistence of fetal hemoglobin, which can ameliorate the clinical severity. , ,
Thalassemia minor or trait is the mildest form and is generally asymptomatic or presents with mild microcytic anemia. Individuals with thalassemia minor are typically heterozygous carriers of a single beta-globin gene mutation, which results in a slight imbalance of globin chain production but does not significantly impair erythropoiesis. On hemoglobin electrophoresis, individuals with thalassemia minor show normal to slightly reduced levels of hemoglobin A, with a mild increase in hemoglobin A2 and occasionally a slight elevation in hemoglobin F. The presence of these alterations in HbA2 and HbF levels in electrophoresis is key for the diagnosis of thalassemia minor, distinguishing it from iron deficiency anemia and other causes of microcytosis. , ,
Anemia in thalassemia arises from a combination of factors, including ineffective erythropoiesis, chronic hemolysis, and increased destruction of abnormal red blood cells. The ineffective erythropoiesis is primarily due to the imbalanced globin chain synthesis, which results in the formation of unstable and dysfunctional hemoglobin, leading to the premature death of erythroid precursors in the bone marrow. This ineffective erythropoiesis leads to a compensatory increase in erythropoietin production and hyperplasia of erythroid precursors in the bone marrow, further exacerbating anemia and bone marrow expansion. Additionally, the destruction of abnormal erythrocytes by the spleen contributes to the severity of anemia in thalassemia patients. , ,
Meanwhile, iron metabolism is profoundly affected in thalassemia, both due to intrinsic disease mechanisms and as a consequence of treatment. Ineffective erythropoiesis in thalassemia leads to increased gastrointestinal iron absorption, even in the absence of iron deficiency, due to the suppression of the iron-regulatory hormone hepcidin. Moreover, patients with moderate to severe thalassemia often require regular blood transfusions to manage their anemia, which introduces additional iron into the body. This transfusion-related iron overload, combined with increased intestinal iron absorption, leads to progressive accumulation of iron in vital organs such as the liver, heart, and endocrine glands, causing significant morbidity and mortality due to complications like liver cirrhosis, diabetes, and IOC. , This part will be extensively discussed in the next section.
Iron overload in thalassemia
Iron overload is a critical complication in thalassemia, resulting from both intrinsic and extrinsic factors that disrupt normal iron homeostasis. The primary mechanisms of iron overload in thalassemia include ineffective erythropoiesis, increased gastrointestinal iron absorption, and frequent blood transfusions. , Ineffective erythropoiesis leads to chronic anemia, which, in turn, suppresses hepcidin production through the mediation of erythroferrone. Hepcidin, a liver-derived hormone, is the key regulator of iron balance in the body. It functions by inhibiting iron absorption from the intestine and iron release from macrophages. In thalassemia patients, low hepcidin levels lead to unregulated iron absorption from the diet, exacerbating iron overload. , Moreover, the frequent blood transfusions required to manage anemia in thalassemia major patients introduce a substantial amount of iron into the body. Each transfusion adds approximately 200-250 mg of iron, leading to a cumulative burden that the body cannot excrete, resulting in systemic iron overload.
The excess iron absorbed and introduced through transfusions is primarily stored in the liver, which is the main organ for iron storage and detoxification. Over time, iron accumulation in the liver can lead to liver fibrosis, cirrhosis, and eventually hepatocellular carcinoma. In addition to the liver, iron also accumulates in other organs, such as the endocrine glands and the heart. , Iron deposition in the endocrine glands can cause dysfunction in the pancreas, leading to diabetes, and affect the pituitary gland, resulting in growth retardation and delayed puberty. , Cardiac iron overload is particularly dangerous, as it can lead to cardiomyopathy, heart failure, and arrhythmias, which are major causes of morbidity and mortality in thalassemia patients. Thus, monitoring and managing iron overload are essential components of thalassemia care.
To assess and monitor iron overload, biomarkers such as serum ferritin and liver iron concentration (LIC) are commonly used. Serum ferritin, an acute-phase reactant, reflects the body’s iron stores, with elevated levels indicating iron overload. However, serum ferritin can be influenced by inflammation and liver disease, making it a less specific marker for iron overload in some cases. Liver iron concentration, measured through liver biopsy or non-invasive imaging techniques like MRI, provides a more accurate assessment of total body iron stores and is considered the gold standard for monitoring iron overload in thalassemia patients.
Emerging biomarkers are being explored to provide more precise and reliable assessments of iron overload. These include hepcidin levels, which could offer insight into the regulation of iron absorption, and soluble transferrin receptor (sTfR) concentrations, which reflect erythropoietic activity and iron demand. Additionally, advanced MRI techniques are being developed to assess cardiac iron load more accurately, providing a valuable tool for predicting and preventing cardiac complications in thalassemia patients. These emerging biomarkers hold the potential to improve the early detection and management of iron overload, ultimately enhancing the quality of life and clinical outcomes for thalassemia patients.
Iron overload cardiomyopathy: mechanisms and pathophysiology
IOC is a significant clinical concern in patients with thalassemia, characterized by excessive iron deposition in the myocardial tissues. This condition is a primary cause of morbidity and mortality in thalassemia patients, leading to heart failure, arrhythmias, and sudden cardiac death. The clinical significance of IOC lies in its insidious onset and progressive nature, often remaining asymptomatic in the early stages until irreversible myocardial damage occurs. Therefore, early detection and intervention are crucial for improving patient outcomes and reducing cardiovascular complications associated with iron overload.
The pathophysiological mechanisms underlying IOC are complex and multifactorial ( Fig. 1 ). Normally, the body tightly regulates iron absorption and storage. The liver hormone hepcidin controls iron entry into the circulation by inhibiting the iron exporter ferroportin. When iron levels are high, hepcidin production increases, limiting iron absorption. However, in thalassemia, reduced hepcidin production leads to unregulated iron absorption from the gut. Once absorbed, non-transferrin bound iron (NTBI) circulates in the blood and deposits in the heart. Moreover, frequent blood transfusions in thalassemia release iron, leading to iron buildup in the heart. The excess iron is stored in cardiomyocytes as ferritin or hemosiderin, but these stores eventually get overwhelmed, leading to the buildup of labile iron, which is highly reactive and generates reactive oxygen species (ROS). Direct iron toxicity on myocardial cells is a critical factor, where excessive labile iron deposits in the heart generate free radicals (i.e., hydroxyl radicals [OH•]) through Fenton reactions. These ROS lead to oxidative stress, damaging cellular components, including lipids (through lipid peroxidation), proteins (via protein oxidation), and DNA, and impairing the function of cardiomyocytes. This oxidative damage disrupts the integrity of cell membranes and organelles (e.g., mitochondria and sarcoplasmic reticulum), leading to cell death and fibrosis, which contribute to the deterioration of cardiac function.
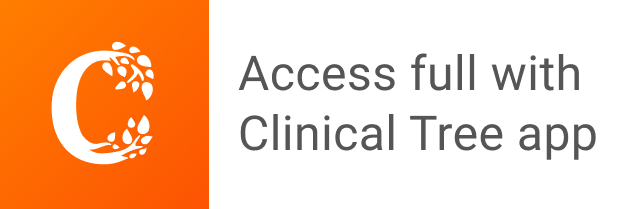