The atrial sites associated with fractionated activity and/or high-frequency signals are commonly considered as targets of ablation for atrial fibrillation (AF); however, their temporal stability has not been established. A total of 21 patients with paroxysmal AF were studied. Left atrial (LA) ganglionated plexi (GP) were identified by high-frequency stimulation, and prolonged (3-minute) electrogram sampling from the GP and the posterior wall of the left atrium during AF was acquired. Fast Fourier transformation was used to determine the dominant frequencies (DFs) of the recorded electrogram signals and to study their temporal variability. The DF at the identified GP was 5.34 ± 0.78 Hz and at the posterior LA wall was 5.58 ± 0.87 Hz. Fractionation, expressed as electrograms exhibiting consecutive DFs deferring >20%, was detected at 21 of the studied GP (84%) and 7 of the posterior LA wall sites (44%). Fractionation, expressed as electrograms exhibiting DFs >8 Hz, was detected at 6 GP (24%) and 1 posterior LA wall site (6%). During the 3-minute recordings, the derived DFs were temporally variable, exhibiting an average coefficient of variation of 15.2 ± 12.0%. Fractionation, expressed by significant consecutive DF variability (>20%), was detected only for 18.0 ± 19.0% of the recording period at GP and for 12.7 ± 13.4% at the posterior LA wall. In conclusion, atrial electrograms are temporarily variable, and fractionation is transient at atrial sites associated with fractionated electrical activity during AF. Our results question the clinical validity of fractionated atrial electrograms for ablation purposes.
Analysis in both time and frequency domains has been used to detect fractionated electrical activity; however, the ideal method has not been established. Complex fractionated atrial electrograms (CFAEs) have been used for the identification of potential ablation targets sites, albeit with variable results. CFAEs can also represent sites of passive wavefront collision at the periphery of high-frequency sources and can be detected in most left atrial (LA) endocardial locations. Thus, additional criteria, such as continuous electrical activity and the detection of a high dominant frequency (DF) of the signal (i.e., DF >8 Hz), have been proposed for identification of fractionation and ablation purposes. The concept of electrogram-based catheter ablation of atrial fibrillation (AF) by identifying sites of complex electrical activity is valid only if these sites are temporally stable. The initial reports using automated algorithms for electrogram analysis in the time domain or in the frequency domain suggested that fractionated electrograms are reproducible and stable over time. However, these studies were hampered by limited sampling times of 2 to 8 seconds or, at the most, 30 seconds. Longer recordings for 60 seconds have revealed a large degree of temporal variation in the electrogram characteristics at certain LA locations. When continuous electrogram analysis during a 5-minute period was considered, significant temporal variability of conventional CFAE maps and sequential DFs was detected. There has also been evidence supporting an association between autonomic activity and the development of electrical fractionation, and CFAEs have been found more prominent in areas that correspond with the anatomic location of ganglionated plexi (GP) in the left atrium. No data on the temporal stability of fractionation recorded at these sites exist. The aims, therefore, of the present study were to assess fractionated activity using spectral analysis at LA locations that have been associated with CFAE and/or high-frequency signals, such as the posterior LA wall and the anatomic sites of GP in patients with paroxysmal AF and to consider temporal stability of the fractionated signals over prolonged acquisition intervals at these LA locations.
Methods
A total of 21 patients (age 52 ± 12 years, LA diameter 41.7 ± 4.1 mm, left ventricular ejection fraction 58 ± 7%) referred for first-time catheter ablation of paroxysmal AF to Athens Euroclinic were enrolled. At study entry, antiarrhythmic medication had been discontinued for 5 half-lives, and no patient was taking amiodarone. Our institutional ethics committee approved the present study, and all patients provided written informed consent.
Mapping in all patients was accomplished by the same experienced operator (D.G.K.). Spiral computed tomography was performed, and the resulting image of the left atrium was integrated with the electroanatomic map of the left atrium (Carto, Biosense-Webster, Diamond Bar, California). LA and pulmonary vein mapping was performed during AF. If the patient was in sinus rhythm, AF was induced by high-rate atrial pacing without the use of isoproterenol. An irrigated-tip ablation catheter with a 3.5-mm tip and an interelectrode spacing of 2, 5, and 2 mm (Cordis-Webster) was used for mapping purposes. The anatomic areas of GP have been previously described: left superior GP, left inferior GP, right anterior GP, and right inferior GP. After anatomic orientation, identification of GP was accomplished by high-frequency stimulation. The patients were deeply sedated with diamorphine and diazepam without intubation, and high-frequency stimulation was delivered with a Grass stimulator at 1,200 beats/min (20 Hz) with a pulse width of 10 ms at 12 to 24 V. A predominant efferent vagal response is defined as the induction of an AV block (>2 seconds) and hypotension or prolongation of the R-R interval by >50% during AF, after a 5-second application of high-frequency stimulation. After identification and mapping of the GP, the mapping catheter was positioned at the LA roof between the right and left superior pulmonary vein and then moved toward the posterior wall ( Figure 1 ). High-frequency stimulation was repeated to detect the existence of GP at this area, and posterior LA sampling was accomplished. Both the LA roof and the posterior LA wall have been associated with high-frequency activity. The exact locations of sampling in the GP and posterior LA are indicated in Figure 1 .
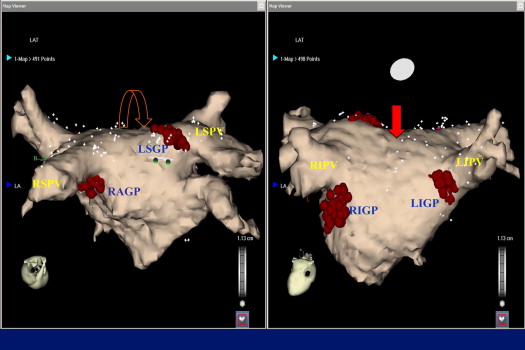
With the catheter in a stable position, electrograms were recorded for 3 minutes (180 seconds) at the anatomic sites of the GPs and the posterior LA wall sites during AF. Care was taken to ensure a stable catheter contact with the endocardium by fluoroscopic visualization of catheter mobility in relation to cardiac motion and by a distance of <2 mm between 2 successive beats as measured using the Carto system. The signals were exported and analyzed off-line with the use of specialized software (Bard FFT dedicated software).
Initial DF analysis was performed using the methods described by Sanders et al. A Hanning window was applied at the signals which were subsequently rectified and band-pass filtered (1 to 20 Hz). A 4,096-point fast Fourier transformation (spectral resolution 0.24 Hz) was used to obtain the power spectrum of the electrogram at each recording site. The fast Fourier transformation was repeated for a sliding window of 2,048 points (2 seconds) until the whole duration of the recording was covered. The 180-second sampling duration with 4,096-point fast Fourier transformation and a 2,048-point sliding window resulted in 84 consecutive fast Fourier transformation computations at each site. In each spectrum, the frequency with the largest amplitude was assigned to be the DF at that site and temporal instant. To ensure reliability in DF detection, we calculated the regularity index. Only points demonstrating a regularity index >0.2 were included in subsequent analysis to control for ambiguity in DF detection related to a poor signal-to-noise ratio. Electrogram fractionation was assessed by 2 approaches: (1) by identifying electrograms at which consecutive DFs deferred >20% during the recording period ; and (2) by identifying electrograms that exhibited a DF >8 Hz during the recording period. The temporal variability of fractionation was assessed by calculating the percentage of the recording period that electrograms were characterized as CFAEs. The overall temporal variability of atrial electrograms was assessed by calculating the coefficient of variation (SD/mean) of the DFs for each recording site.
Statistical analysis
The normality of data distribution was determined using the Kolmogorov-Smirnov test. Data are reported using descriptive statistics (mean ± SD). To further describe the dispersion of measurements, the coefficient of variation of the DF was calculated, defined as the ratio of the SD to the mean. Analyses were performed with parametric or nonparametric methods depending on data distribution. Student’s t test was used to compare the mean of 2 groups and 1-way analysis of variance to compare the mean values of >2 groups. Friedman’s test was applied to estimate the overall significance, and Wilcoxon’s rank sum test was used for pairwise comparisons. p Values were adjusted for multiple comparisons with Holm’s sequential Bonferroni method. Statistical analyses were performed using SPSS Statistics, version 20.0 (IBM, Armonk, New York, New York). All tests were 2-tailed, and adjusted p values <0.05 were considered significant.
Results
Of the 21 patients, 5 could not tolerate high-frequency stimulation, and the experimental procedure was abandoned. Thus, 16 patients were included in the analysis. At least 1 GP could be identified by high-frequency stimulation in all patients, and 2.4 ± 0.8 bipolar electrograms were recorded per patient. No GP was identified by high-frequency stimulation in the posterior LA wall. The identified GPs and corresponding DFs at the GPs and posterior atrial wall are listed in Table 1 . The difference between the DFs at the GPs and the posterior LA wall was not statistically significant (p = 0.511). The DFs calculated for the identified GP were not significantly different (p = 0.204).
Pt. No. | DF (Hz) | ||||
---|---|---|---|---|---|
LSGP (n = 10) | LIGP (n = 4) | RAGP (n = 3) | RIGP (n = 8) | Posterior LA Wall (n = 16) | |
1 | 5.21 ± 0.25 | 5.04 ± 0.39 | 5.11 ± 0.42 | ||
2 | 5.58 ± 0.35 | 5.52 ± 0.43 | |||
3 | 5.13 ± 0.46 | 4.07 ± 1.35 | 5.41 ± 0.28 | 4.89 ± 1.23 | |
4 | 5.14 ± 0.73 | 4.84 ± 0.52 | |||
5 | 4.66 ± 1.46 | 6.71 ± 1.55 | |||
6 | 4.24 ± 1.85 | 5.51 ± 0.45 | |||
7 | 5.71 ± 0.33 | 5.60 ± 0.34 | |||
8 | 5.11 ± 0.71 | 5.31 ± 0.72 | 5.14 ± 0.53 | ||
9 | 6.19 ± 0.28 | 6.52 ± 1.18 | 6.40 ± 0.27 | ||
10 | 7.04 ± 1.12 | 6.42 ± 0.38 | 7.04 ± 0.30 | ||
11 | 5.39 ± 0.22 | 4.89 ± 0.69 | 5.17 ± 0.14 | ||
12 | 4.34 ± 1.92 | 5.43 ± 0.70 | 5.10 ± 0.55 | ||
13 | 6.97 ± 1.48 | 6.44 ± 0.74 | |||
14 | 5.22 ± 1.70 | 4.06 ± 0.82 | 5.78 ± 0.44 | ||
15 | 4.39 ± 1.12 | ||||
16 | 5.79 ± 0.33 | 4.55 ± 0.59 | 5.65 ± 0.22 |
Fractionation, expressed as electrograms exhibiting consecutive DFs deferring >20%, was detected at 21 of the GPs (84%) and 7 of the posterior LA wall sites (44%). Fractionation, expressed as electrograms exhibiting DFs >8 Hz, was detected at 6 GPs (24%) and 1 posterior LA wall site (6%). All electrograms with DFs >8 Hz at particular instances also exhibited consecutive DFs that deferred >20%. Fractionation at the sampled sites was not temporally stable; at GP fractionation, expressed as electrograms exhibiting significant consecutive DF variability (>20%), was detected only for 18.0 ± 19.0% of the recording period. At the posterior LA wall, it was detected for 12.7 ± 13.4% of the recording period. This is illustrated in Figure 2 , in which the variation of consecutive DFs and the detection of CFAE with time are shown for electrograms acquired from the right inferior GP and the LA posterior wall. Although the temporal variability of fractionation was more marked at the GPs compared with the posterior left atrium, the difference between the 2 sites was not statistically significant (p = 0.295).
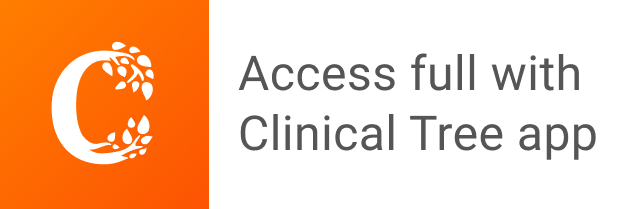